Perspectives on hydrogel-based ionic thermoelectrics: from mechanistic insights to wearable applications of thermo-diffusive ionic materials
Abstract
Hydrogel-based ionic thermoelectric (i-TE) materials that rely on ion migration driven by thermal gradients have emerged as promising candidates for efficient low-grade heat harvesting. They offer high Seebeck coefficients, mechanical flexibility, and biocompatibility, making them especially attractive for wearable electronics and biomedical applications. Among various i-TE materials, hydrogels are particularly notable due to their unique structure and ability to modulate ion diffusion via interactions between the polymer network and ionic species. Despite increasing interest in hydrogel-based i-TE materials, the fundamental mechanisms governing thermodiffusive ion transport remain poorly understood, especially when compared to the more established thermo-galvanic processes. Moreover, the unique composite architecture of these materials combining polymer matrices with diverse ionic components presents significant challenges for rational design and performance optimization. This review addresses these challenges by systematically analyzing the fundamental mechanisms of hydrogel-based i-TE materials, with a particular focus on the Soret effect and the roles of polymer networks and ionic conductors. It also provides critical insights into practical applications such as wearable thermoelectric generators and capacitive energy storage devices. Furthermore, we propose innovative strategies to overcome key limitations, those related to long-term stability and mechanical durability. By consolidating current knowledge and identifying future research opportunities, this review establishes a foundation for the development of next-generation flexible and efficient hydrogel-based i-TE materials.
Keywords
INTRODUCTION
Energy harvesting from waste heat has become an important strategy for improving energy efficiency, with growing interest in its application to flexible and wearable electronics[1-3]. Among various energy conversion technologies, thermoelectric (TE) materials offer a direct means of converting thermal energy into electrical energy through the Seebeck effect, which relies on the movement of charge carriers under a temperature gradient[4]. However, conventional TE materials face challenges in harvesting low-grade heat (< 100 °C), which is typically dissipated under realistic environmental conditions, due to drawbacks such as rigidity, toxicity, and relatively low Seebeck coefficients in the tens of μV K-1 range[5,6]. These challenges have driven the search for alternative TE materials capable of efficiently converting low-grade heat into electrical energy[7-10]. Notably, waste heat released in daily life mainly originates from non-planar surfaces such as the human body. To achieve effective heat-to-electricity conversion from such curved heat sources, next-generation TE materials are required with (i) high energy conversion efficiency near room temperature[11-13]; (ii) adaptable mechanical flexibility to conform to curved geometries[14,15]; and (iii) sustainable stretchability to accommodate abrupt movements[16-18].
Ionic thermoelectric (i-TE) materials have emerged as a promising alternative to traditional e-TE materials due to their unique combination of high Seebeck coefficient, mechanical flexibility, biocompatibility, and processability[19,20]. Unlike e-TE materials, i-TE materials convert thermal energy into electrical energy under a temperature gradient through two primary mechanisms: the Soret effect[21] and the thermo-galvanic effects[22]. Soret-effect-based i-TE materials have recently gained attention due to their high Seebeck coefficient and ability to maintain a stable potential difference without involving redox reactions. The Soret effect refers to the phenomenon where a thermal gradient induces the migration of cationic and anionic ions with different mobilities, leading to charge separation and the generation of an electric potential difference at the ends[23]. Notably, i-TE materials can exhibit Seebeck coefficients in the millivolt-per-Kelvin range owing to the entropy difference between cations and anions at different temperatures[24]. This characteristic makes them well-suited for harvesting low-grade heat, including body heat, in wearable electronics[21,25,26].
The i-TE materials can be categorized into three types based on their physical states: solid-state, liquid-state, and quasi-state[27]. Hydrogels, a promising class of quasi-state i-TE materials, consist of a three-dimensional polymer network filled with water and ionic conductors. A key advantage of i-TE hydrogels is their intrinsic tunability, allowing their structural and compositional components to be tailored for specific performance requirements[28-30]. This tunability enables precise control over mechanical robustness and flexibility, while facilitating synergistic interactions among the polymer, water, and ions[31]. These features synergistically enhance TE performance by promoting ionic mobility and selectivity, establishing hydrogel as a versatile platform for diverse TE applications. Their diverse mechanical properties allow them to conform to irregular surfaces, ensuring intimate thermal contact with the skin, which makes them highly suitable for wearable applications[32-34]. Furthermore, their inherent biocompatibility enables integration into biomedical devices, such as implantable energy harvesters and self-powered biosensors. The unique combination of high stretchability, biocompatibility and excellent ionic conductivity make hydrogels highly attractive for wearable applications.
Hydrogel-based i-TE materials primarily consist of two key components: the polymer matrix and the ionic conductor, whose composition directly determines TE performance [Figure 1][35-40]. The polymer matrix not only provides mechanical support but also facilitates ion transport by influencing interactions between polymers and ion conductors such as salts and ionic liquids, to regulate the diffusion ratio between both ends[41,42]. The hydrogel matrices in i-TE materials can be broadly classified into natural and synthetic polymers. Natural polymers, such as cellulose, offer sustainability, biocompatibility, and a hierarchical structure that enhances ion transport[30,43]. In contrast, synthetic polymers, such as polyvinyl alcohol (PVA) and polyacrylamide (PAM), provide tunable mechanical properties, improved durability, and chemical stability, allowing optimization for specific applications[44-47]. The hydrogen-bonded networks of polymers can be engineered to influence ion mobility and TE response[48]. Increasing the density of negatively charged functional groups within the polymer matrix enhances cation mobility, resulting in positively charged ions serving as the dominant charge carriers, which favors p-type behavior. Conversely, introducing strongly bound cations that suppress their mobility promotes anion transport, leading to the predominance of negatively charged ions and, consequently, n-type behavior. Another key advantage of hydrogel-based i-TE materials is their capability to facilitate selective ion transport, which determines whether the material exhibits p-type or n-type behavior[29,49,50]. The transport of specific ions can be controlled by adjusting the polymer composition, incorporating functional groups, or selecting specific ionic species. This tunability is a defining feature of hydrogel-based i-TE materials, offering versatile design strategies for optimizing TE performance[36,47]. Beyond polymer selection, the choice of ionic conductor also significantly impacts TE efficiency. Salts such as LiCl, NaCl, and KCl provide high ionic conductivity and a substantial Seebeck coefficient at low cost. In contrast, ionic liquids (ILs), which are low-volatile organic salts, offer superior thermal and chemical stability, ensuring sustained TE performance over extended periods. The intricate interactions among the polymer matrix, water content, and ionic species significantly impact energy conversion efficiency by governing both the Seebeck coefficient and overall conductivity[27,51,52]. Therefore, the rational design of hydrogel-based i-TE materials is essential to achieve structural stability, optimized ion transport, and enhanced TE performance.
Figure 1. Overview of hydrogel-based ionic thermoelectric (i-TE) materials. Reproduced with permission[35-40]. Copyright 2024, Elsevier Ltd.; Copyright 2025, Royal Society of Chemistry; Copyright 2023, Elsevier Ltd.; Copyright 2024, Elsevier Ltd.; Copyright 2022, Elsevier Ltd.; Copyright 2023, Wiley-VCH GmbH.
This review aims to provide a comprehensive understanding of hydrogel-based i-TE materials, covering aspects ranging from their mechanical properties to wearable applications, with a particular focus on the intermolecular interactions between polymers and ion conductors. By examining their fundamental mechanisms, material compositions, and performance optimization strategies, this review provides important factors influencing TE efficiency. Three types of polymers - cellulose, PVA, and PAM - are discussed in terms of their roles in facilitating selective ion transport, along with the impact of salt-based and IL-based electrolytes on TE performance. By elucidating these relationships, this review offers valuable insights into rational material design and presents potential strategies for enhancing TE efficiency. Additionally, it systematically analyzes polymer matrices, ionic conductors, and their interactions to evaluate TE performance based on the type of salts and ILs. This chapter aims to establish design principles for the development of next-generation i-TE materials to address critical energy challenges in wearable electronics, biomedical devices, and sustainable power generation, as well as to overcome major challenges for practical applications, including temperature and humidity stability and mechanical durability.
BASIC PRINCIPLE
Key parameters of TE performance in hydrogel-based thermoelectric systems
The performance of TE materials is commonly assessed using the dimensionless figure of merit (ZT), which is defined as[53]:
where S represents the Seebeck coefficient, σ denotes the electrical conductivity, and κ signifies the thermal conductivity [Figure 2A and B]. Z represents the TE material parameter, which quantifies the intrinsic ability of a broad range of TE materials to convert thermal energy into electrical energy. T is the absolute temperature at which the material operates, playing a critical role in determining TE efficiency. Since
Figure 2. (A) Thermoelectric figure of merit (ZT) and its key parameters, including the Seebeck coefficient (S), electrical conductivity
i-TE energy conversion primarily operates through two mechanisms: the thermodiffusion effect (Soret effect) and the thermo-galvanic effect [Figure 2C and D]. While both mechanisms contribute to ion-based TE energy conversion, the thermo-galvanic effect relies on redox reactions at the electrodes, which can induce kinetic limitations and long-term stability issues. In contrast, the thermodiffusion effect drives ion migration under a temperature gradient, ensuring stable and continuous energy conversion without any chemical reactions[56]. Since hydrogel-based i-TE materials with thermodiffusion effect achieve tunable selective ion migration and facilitate directional ion transport, they can reduce energy dissipation and improve processability for wearable applications. Given these trends, we discuss the fundamental working principle of hydrogel-based i-TE materials, specifically focusing on the Soret effect rather than the thermo-galvanic effect in this section.
Ion diffusion and ion migration in hydrogel-based thermoelectric systems
Thermodiffusion (Soret effect) refers to the ion diffusion process induced by a temperature gradient. Differences in ion diffusion rates driven by thermal gradient leads to the formation of an ion concentration gradient, generating electrochemical potential differences[56]. This mechanism differs from conventional e-TE materials, which induce charge transport through electron and hole movement[57]. When a hydrogel containing a dissolved electrolyte is subjected to a temperature gradient (∇T), cations and anions diffuse at different rates depending on their hydration energy and mobility. This dissymmetric diffusion results in charge accumulation at opposite ends of the hydrogel, inducing a potential difference. To understand ion transport under a temperature gradient, the absence of any external force is assumed. Under this assumption, the ion flux (Ji) in i-TE materials can be expressed by[56]:
where D is the diffusion coefficient, DT is the thermodiffusion coefficient, ni is the ion concentration, and T is the absolute temperature. The Eq. 1 describes ion transport driven by both a concentration gradient (kinetic diffusion; first term) and a temperature gradient (thermodiffusion; second term). At equilibrium state (Ji = 0) with no external force, the thermodiffusion-induced potential difference follows[56]:
The Soret effect can be denoted as:
where DT/D = St is the Soret coefficient. The Soret coefficient describes the formation of an ion concentration gradient in response to temperature gradient. If cations and anions exhibit different Soret coefficients, a local net charge distribution will form under a temperature gradient, thereby generating an electric potential (∇V).
where ST represents the Seebeck coefficient of the hydrogel-based i-TE system. In the hydrogel-based i-TE system, a higher ST indicates greater thermodiffusion-induced charge separation, leading to increased potential difference under a given temperature gradient. In addition, the ion total flux (J) under steady state condition (
where JD is the diffusive flux, JE is the electromigrative flux, JT is the thermodiffusive flux, qi is the charge of the ion,
According to Onsager transport theory and assuming symmetric electrolytes (q+ = q- and n0+ = n0-), ST can be simplified as[21,61]:
where the n+ and n- represent the concentrations of cations and anions, respectively, and e is the electron charge. Therefore, the Seebeck coefficient of i-TE materials is higher than that of e-TE materials, which can be attributed to the difference in Eastman entropy inherent in their TE processes[61,62]. In i-TE materials, Eastman entropy is influenced by interactions between ions and their surrounding environments, such as matrix, solvent and other ions[52]. Consequently, the ST of hydrogel-based i-TE systems is highly dependent on the ionic species, hydrogel network structure, and hydration state. In addition, if the thermal mobility and ionic mobility are defined using Einstein’s relation, they can be expressed as follows, respectively[21]:
Considering the μT and μi, the ST can be expressed as[21]:
Furthermore, assuming that the hydrogel has homogeneous system (n+ = n-), the ST can be expressed as[56]:
As a result, the ST is dominated by the difference in thermodiffusive mobility between cations and anions. A greater disparity in thermodiffusive mobility further enhances the TE performance of i-TE materials.
Effects of water molecules on ion mobility and Seebeck coefficient
The TE performance of hydrogel-based materials is significantly influenced by their water content, which directly affects ion mobility and overall charge transport efficiency. Water serves as a primary medium for ion transport, facilitating efficient charge transport through hydrated pathways. The ionic conductivity (σ) of hydrogel is expressed as[63]:
An increase in water content generally enhances ion mobility by reducing frictional resistance and enabling more efficient ion diffusion[64]. However, excessive hydration can dilute ion concentration, leading to a lower charge carrier density and reduced overall conductivity. Thus, maintaining an optimal water balance is crucial for achieving high-performance hydrogel-based TE systems.
Water content also directly influences ionic thermo-diffusion and overall TE performance[65]. In addition, water molecules influence thermal management within hydrogel-based i-TE materials. Hydrogels typically exhibit lower thermal conductivity (κ) compared to solid-state materials[63]. Their high specific heat capacity attributed to water content prevents localized overheating and ensures uniform temperature gradient across the material. Furthermore, hydrogels with hygroscopic properties can absorb moisture from the surrounding environment, enabling simultaneous water evaporation and absorption. This feature serves as an additional cooling mechanism, especially beneficial for applications requiring continuous contact with both the body and ambient air.
COMPONENTS OF IONIC THERMOELECTRIC HYDROGEL
Hydrogels, a class of soft materials, consist of a water-filled polymer network that provides both mechanical flexibility and processability. These characteristics make hydrogels particularly attractive as i-TE materials, which exhibit a quasi-solid-state nature combining both solid-like and fluid-like properties. A crucial component of hydrogels for i-TE applications is the ionic conductor, which facilitates ion migration and enhances TE performance[21,33,41]. Intrinsically, hydrogels contain water that undergoes autoionization to produce H3O+ and OH- ions, enabling some degree of TE activity[66]. However, the resulting Seebeck coefficient is relatively low and insufficient for practical applications. To overcome these issues, researchers have designed hydrogels to incorporate high-potential ionic conductors, such as salts and ILs, within the polymer matrix to enhance ion motility and concentration. The overall TE performance of i-TE hydrogels is governed by the interactions between the polymer networks and the ionic conductors. The polymer network serves as a structural framework that ensures mechanical integrity and modulates ion transport pathways. In parallel, the ionic conductor governs the Seebeck coefficient and charge carrier type (p-type or n-type) through polymer-ion interactions and ion mobility within the hydrogel matrix. Since the Seebeck coefficient is closely linked to thermally driven ion diffusion, understanding these interactions is crucial for optimizing i-TE material design[64,67-71]. This section explores the key polymeric materials commonly used as i-TE hydrogels, followed by an in-depth discussion on different ionic conductors, including salts and ILs, to elucidate their roles in governing TE performance and charge transport mechanisms.
Polymer networks
The polymer network is a fundamental component of i-TE hydrogels, providing both mechanical integrity and interconnected pathways for ion diffusion, which directly influences TE performance. Natural polymers such as cellulose, gelatin, and chitosan offer biocompatibility, renewability, and environmental sustainability, making them suitable for eco-friendly and biomedical wearable applications[39,65]. In particular, cellulose is widely used due to its porous structure that facilitates ion conduction. In contrast, synthetic polymers such as PVA and PAM provide high structural tunability and mechanical stability, allowing for tailored material optimization. PVA forms strong hydrogen bonds with water through its hydroxyl (-OH) groups, resulting in excellent water retention, flexibility, and chemical stability[46,50]. Similarly, PAM possesses amide groups (-CONH2) that enhance water retention and ionic conductivity, serving as an effective platform for i-TE applications[72]. This section focuses on the representative polymeric materials used in i-TE hydrogels, specifically cellulose as a natural polymer and PVA and PAM as synthetic polymers. Each material exhibits unique physical and chemical characteristics that influence their interaction with ionic conductors through ion-matrix-water interactions. This section provides a detailed analysis of their roles in optimizing ion mobility, charge selectivity, and overall TE efficiency, along with strategies for engineering them to enhance i-TE performance.
Cellulose
Cellulose, a naturally derived polymer, is widely utilized in i-TE materials due to its renewability, biocompatibility, and ionic conductivity. Consisting of glucose monomers with hydroxyl (-OH) groups, cellulose forms a hydrogen-bonded network that provides mechanical stability and efficient ion transport[73]. Its hierarchical fibril structure promotes anisotropic ion diffusion, while surface-exposed negatively charged oxygen species (O-) selectively facilitate cation hopping, favoring p-type behavior. Chemical modifications, such as the incorporation of carboxyl (-COO-) or sulfonate (-SO3-) groups, further enhance ion selectivity and mobility. Additionally, the addition of salts or ILs optimizes thermally induced ion transport, improving the Seebeck coefficient.
For p-type i-TE materials, cation-dominated transport mechanisms play a critical role. By leveraging the inherent negative surface charge of cellulose and optimizing ion-polymer interactions, researchers have developed efficient strategies to enhance cation transport through structural modifications. One key strategy involves engineering cellulose to form anion-repelling nanochannels. Li et al. introduced negatively charged surfaces in cellulose nanofibers to attract Na+ ions while repelling OH-, thereby significantly increasing ion selectivity [Figure 3A][30]. The optimized NaOH-cellulose electrolyte facilitated ion mobility through well-ordered nanochannels, achieving a thermal voltage of 24 mV K-1, more than twice that of conventional systems. Hu et al. further improved p-type properties by incorporating quaternary ammonium-based electrolytes, BzMe3NOH into the cellulose matrix[74]. This system yielded a Seebeck coefficient of
Figure 3. Cellulose-based i-TE materials and their TE properties. The figure includes the structural representation of cellulose and its role in i-TE materials, highlighting key TE properties such as ion transport and Seebeck coefficient. (A) Schematic illustration of the enhanced ion mobility and selectivity of the cellulosic membrane due to the nanochannels formed between the cellulose nanofibres and the effect of intermolecular bonding. Reproduced with permission[30]. Copyright 2019, Springer Nature; (B) Schematic diagrams of thermovoltage generation in cellulose/BzMe3NOH hydrogel. (C) Seebeck coefficient of cellulose/BzMe3NOH hydrogel with various
While cellulose-based p-type i-TE materials have been extensively studied, efforts to develop n-type i-TE materials remain at an early stage. Achieving n-type behavior requires promoting anion transport while suppressing cation transport. This is typically accomplished by modifying the cellulose matrix to increase its negative surface charge density, which immobilizes cations and facilitates anion diffusion. Liu et al. demonstrated an n-type cellulose system by incorporating [BMIM][BF4] into a cellulose-nanofiber (CNF) with poly(acrylamide) (PAM) hydrogel[75]. Strong interactions between the BMIM+ cations and the CNF component restricted cation mobility, allowing BF4- anions to dominate thermodiffusion. Optimizing the [BMIM][BF4] concentration resulted in the highest anionic diffusion efficiency, while the CNF improved an ionic conductivity to 13.9 mS cm-1. Wu et al. improved n-type cellulose by introducing Ca2+ ions into bacterial cellulose (BC) nanochannels. These ions strongly interacted with BC molecular chains to immobilize Na+ while facilitating Cl- diffusion. The system achieved a Seebeck coefficient of -27.2 mV K-1 and an ionic conductivity of 204.2 mS cm-1 [Figure 3D-F][43].
These studies highlight the versatility of cellulose as a platform for both p-type and n-type i-TE materials, emphasizing the importance of surface charge modulation and polymer-ion interactions in achieving selective ion transport. Tailoring polymer-ionic conductor interactions enables enhancements in ionic conductivity, surface charge density, and molecular channel architecture, thereby expanding the design possibility for high-performance cellulose-based i-TE materials. A summary of recent advancements in cellulose-based i-TE hydrogels is presented in Table 1[30,35,37,40,43,47,50,74-77].
TE performance of i-TE hydrogels with cellulose networks
Authors | Polymers | Ion conductors | Type | Si [mV/K] | σi [mS/cm] | κi [W/mK] |
Li et al.[30] | Cellulose | NaOH | p | 24 | 20 | 0.48 |
Qian et al.[35] | CMC PAM | LiCl | p | 2.96 | 36.51 | 0.575 |
Chen et al.[37] | CBC | LiTFSI | p | 11.5 | 0.71 | - |
Han et al.[40] | PQ-10 | NaOH | p | 24.2 | 0.3 | - |
Yang et al.[47] | CMC PAA | NaCl | p | 17.1 | 26.8 | - |
Hu et al.[74] | Cellulose | BzMe3NOH | p | 2.61 | 38 | 0.18 |
Cheng et al.[76] | Cellulose | KCl | P | 22.1 | 0.64 | 0.31 |
Wu et al.[43] | BC | NaCl | n | -27.2 | 204.2 | - |
Chen et al.[50] | Cellulose PVA | [CuCl4]2- | n | -26.3 | 8.47 | 0.47 |
Liu et al.[75] | Cellulose PAM | [BMIM][BF4] | n | -20.1 | 13.9 | 0.46 |
Chen et al.[77] | BC PVA | NaOH | n | -20.7 | 0.62 | - |
Polyvinyl alcohol
Polyvinyl alcohol (PVA) is a widely studied synthetic polymer for i-TE materials, exhibiting p-type behavior due to its strong affinity for cations. This characteristic arises from its abundant hydroxyl (-OH) groups, which enable extensive hydrogen bonding, stabilize the hydrogel matrix, and modulate both ionic diffusion pathways and water retention[78]. Partial ionization of these hydroxyl groups generates negatively charged sites that selectively attract cations, thereby reinforcing p-type conduction. The incorporation of salts or ILs further promotes thermally induced ion transport, leading to an enhanced Seebeck coefficient. These structural advantages make PVA a promising candidate for flexible and bio-integrated TE devices.
Recent studies have focused on optimizing cation-dominated transport to enhance the TE performance of PVA-based materials. By utilizing the high density of hydroxyl groups and strong hydrogen bonding, researchers have developed strategies to improve cation selectivity and mobility. Chen et al. enhanced H+-dominated thermodiffusion in PVA hydrogels by reinforcing hydrogen bonding and crystallinity through tensile crystallization [Figure 4A-C][79]. This approach selectively enhanced H+ mobility while restricting Cl- diffusion, achieving a Seebeck coefficient of 38.20 mV K-1, twice that of conventional materials. Similarly, Hsiao et al. designed a double-network PVA/sodium alginate (SA)/polyethylene glycol (PEG) hydrogel, incorporating NaBF4 to optimize Na+ transport while limiting BF4- mobility[45]. The synergistic effects of counter-ion condensation and PVA crystallinity resulted in a high Seebeck coefficient of 66.7 mV K-1 and an ionic conductivity of 31.4 mS cm-1, along with a power factor of 13.96 mW m-1K-2 [Figure 4D-F][45]. These studies demonstrate the effectiveness of structural modifications and crystallization in enhancing ion-polymer interactions and ionic Seebeck coefficients of PVA-based p-type i-TE materials.
Figure 4. PVA-based p-type i-TE materials and their TE properties. The figure includes the structural representation of PVA and illustrates its interactions within the hydrogel network, highlighting the relationship between structure and TE performance such as ion transport and the Seebeck coefficient. (A) Structural illustration of a TE system enhanced by hydrogen ion interactions within a strong hydrogen bond system. (B) Diffusion coefficients of different ions in aqueous solution. (C) Seebeck coefficient of HCl, HNO3, and H2SO4 in aqueous solution and PVA hydrogels. Reproduced with permission[79]. Copyright 2022, American Chemical Society; (D) Schematic illustration of interatomic interactions between ions and PVA. (E) Increase in -ΔV as a function of ΔT, representing the Seebeck coefficient, and (F) Ionic conductivity as a function of NaBF4 concentration. Reproduced with permission[45]. Copyright 2023, Elsevier Ltd.
While PVA primarily exhibits p-type behavior, it can also be engineered to exhibit n-type properties by enhancing anion mobility while restricting cation diffusion. This is typically achieved by increasing the negative surface charge density, incorporating polyelectrolytes, or modifying the polymer network to selectively facilitate anion transport. Li et al. developed an n-type PVA hydrogel with sulfonate (-SO3-) functional groups, forming a hierarchical polymer network that facilitated Cl- transport while immobilizing K+ through interactions with -OH and -SO3- groups [Figure 5A-C][36]. Additionally, expanding the PVA chains using dimethyl sulfoxide (DMSO) optimized ion selectivity, resulting in a Seebeck coefficient of
Figure 5. PVA-based n-type i-TE materials and their TE properties. The figure includes the structural representation of PVA and illustrates its interactions within the hydrogel network, highlighting the relationship between structure and TE performance. (A) Design schematic of PVA modified by sulfonate (-SO3-). (B) Increase in -ΔV as a function of ΔT, representing the Seebeck coefficient. (C) Ionic conductivity (σi), thermal conductivity (κ), power factor (PFi) and figure of merit (ZTi) of hydrogel electrolytes. Reproduced with permission[36]. Copyright 2025, Royal Society of Chemistry; (D) Schematic illustration of the fabricated i-TE materials based on coordination interaction between Na+ and -OH groups of NaOH-PVA hydrogels under the temperature gradient. Seebeck coefficient of fabricated PVA hydrogels (E) before and (F) after dry-annealing process with different NaOH concentrations. Reproduced under the terms of the Creative Commons CC-BY license[29]. Copyright 2021, American Association for the Advancement of Science.
TE performance of i-TE hydrogels with PVA networks
Authors | Polymer | Ion conductor | Type | Si [mV/K] | σi [mS/cm] | κi [W/mK] |
Hsiao et al.[45] | PVA PEG | Na[BF4] | p | 66.7 | 31.4 | - |
Chen et al.[79] | PVA | HCl | p | 38.2 | 1.89 | 0.46 |
Horike et al.[80] | PVA | [Rmim]Cl | p | 10 | 0.16 | 0.29 |
Chen et al.[29] | PVA | NaOH | n | -37.6 | 0.00736 | 0.42 |
Li et al.[36] | PVA | KCl | n | -16.8 | 17.7 | 0.5 |
Chen et al.[50] | PVA cellulose | [CuCl4]2− | n | -26.3 | 8.47 | 0.47 |
Chen et al.[77] | PVA BC | NaOH | n | -20.7 | 0.62 | - |
Hu et al.[81] | PVA PVP | ZnSO4 | n | -3.69 | 0.15 | - |
Polyacrylamide
Polyacrylamide (PAM) is a hydrophilic synthetic polymer used in i-TE hydrogels due to its high water retention, mechanical flexibility, and tunable ionic interactions. The amide (-CONH2) groups in PAM contribute to hydrogen bonding, stabilizing the hydrogel structure and influencing ionic mobility[82]. These molecular interactions enable adjustable mechanical properties and ionic conductivity, making PAM suitable for both p-type and n-type i-TE applications. Incorporating salts, polyelectrolytes, or ILs further modulates ion diffusion, thus optimizing Seebeck coefficients. Due to its flexibility, hydration stability, and strong ion-polymer interactions, PAM-based hydrogels present a promising platform for next-generation i-TE materials.
PAM-based hydrogels are often optimized for p-type i-TE applications by enhancing selective cation transport [Figure 6A][83]. A major strategy involves introducing negatively charged functional groups, such as carboxyl (-COO-), phosphate (-PO42-), or sulfonate (-SO3-), to increase surface charge density and promote cation diffusion (e.g., Na+, K+, H3O+), thereby improving the Seebeck coefficient. Blending PAM with polymers such as PVA or cellulose nanofibers (CNF) reinforces hydrogen bonding, stabilizes ion transport pathways and improves ionic selectivity. Zhang et al. developed a gelatin/PAM double-network hydrogel that utilized gelatin’s temperature-responsive adhesion and the hydrophilic network of PAM to regulate ion transport [Figure 6B and C][39]. Incorporating LiCl and Li2SO4 enhanced ion selectivity by immobilizing Li+ via amide (-CONH2) and carboxyl (-COO-) coordination, facilitating Cl- diffusion and yielding a Seebeck coefficient of 10.4 mV/K. Similarly, Dai et al. optimized PAM/LiTFSI hydrogels by leveraging Li+-amide (-CONH2) coordination to stabilize Li+ while enhancing TFSI- mobility, achieving a Seebeck coefficient of 19.02 mV K-1 and an ionic conductivity of 2.1 S m-1 [Figure 6D-G][38]. Structural engineering, such as high cross-linking density and ion-polymer coordination, are crucial for optimizing p-type PAM hydrogels.
Figure 6. PAM-based p-type i-TE materials and their TE properties. The figure includes the structural representation of PAM and illustrates its interactions within the hydrogel network, highlighting the relationship between structure and TE performance. (A) Schematic representation of PAM networks in hydrogels and their interactions with ion conductors. Reproduced with permission[83]. Copyright 2022, Royal Society of Chemistry; TE properties of gelatin/polyacrylamide ionic conductive hydrogel: (B) Ionic conductivity and Seebeck coefficient as a function of Li+ concentration. (C) Ionic conductivity and power factor as a function of temperature. Reproduced with permission[39]. Copyright 2022, Elsevier Ltd; Ionic TE properties of PAM/LiTFSI4 hydrogels: (D) Increase in -ΔV as a function of ΔT, representing the Seebeck coefficient. (E) Ionic conductivity, (F) Thermal conductivity, and (G) Ionic ZT as a function of temperature. Reproduced with permission[38]. Copyright 2024, Elsevier B.V.
To achieve n-type behavior in PAM-based i-TE hydrogels, anion transport must be promoted while suppressing cation diffusion. This can be accomplished by incorporating polyelectrolytes such as polystyrene sulfonate (PSS) or ILs, which create conduction channels for anions while immobilizing cations within the polymer network. Strengthening the hydrogen bonding network increases structural density, further restricting cation movement and promoting anion selectivity. Additionally, functionalizing PAM with negatively charged groups, such as carboxyl (-COO-) or sulfonyl (-SO3-), increases surface charge density, thus improving anion diffusion. These strategies enhance the Seebeck coefficient by facilitating the transport of anions such as Cl- and BF4- under a thermal gradient. Jia et al. developed an n-type PAM/ZnSO4 hydrogel in which hydration interactions between Zn2+ and PAM matrix restricted Zn2+ mobility while enhancing SO42- diffusion[64]. This achieved a Seebeck coefficient of -3.72 mV K-1 and an ionic conductivity of 40.44 mS cm-1 [Figure 7A-C][64]. Sha et al. designed a SA-based n-type PAM hydrogel cross-linked with FeCl3, wherein strong interactions immobilized Fe3+ cations and facilitated Cl- transport, yielding a Seebeck coefficient of -2.01 mV K-1 and an ionic conductivity of 1.70 mS cm-1 [Figure 7D-F][84]. Ion-polymer coordination can be strategically employed to tune PAM hydrogel for either p-type or n-type behavior, thereby optimizing their TE performance. Further research on i-TE hydrogels with PAM networks is summarized in Table 3[35,38,39,64,71,75,83-86].
Figure 7. PAM-based n-type i-TE materials and their TE properties. The figure includes the structural representation of PAM and illustrates its interactions within the hydrogel network, highlighting the relationship between structure and TE performance. TE properties of PAM hydrogel-based i-TE materials with various zinc salts and concentrations: (A) Seebeck coefficient, (B) Ionic conductivity, and (C) Power factor (PF). Reproduced with permission[64]. Copyright 2024, American Chemical Society; TE performance of double-network PAM and sodium alginate (SA) hydrogel: (D) Output voltages as a function of filler content and time. (E) Comparison of Seebeck coefficient and ionic conductivity. (F) Voltage response of PAM/SA hydrogel under temperature gradients with a stepwise increase of 5 K. Reproduced with permission[84]. Copyright 2024, Elsevier B.V.
TE performance of i-TE hydrogels with PAM networks
Authors | Polymer | Ion conductor | Type | Si [mV/K] | σi [mS/cm] | κi [W/mK] |
Qian et al.[35] | PAM CMC | LiCl | p | 2.96 | 36.51 | 0.575 |
Dai et al.[38] | PAM | Li[TFSI] | p | 19.0 | 2.1 | 0.37 |
Zhang et al.[39] | PAM Gelatin | LiCl | p | 10.4 | 8.3 | |
Zhou et al.[71] | PAM | CaCl2 | p | 9.31 | ||
Jiang et al.[83] | PAM PDDA | CaCl2 | p | 3.35 | 1.16 | |
Chen et al.[85] | PAM CA | Li2SO4 | p | 11.5 | 10.72 | 0.5085 |
Liu et al.[86] | PAM PEG Alginate | [EMIM][BF4] | p | 19.32 | 12.5 | 0.53 |
Jia et al.[64] | PAM | ZnSO4 | n | -3.72 | 40.44 | |
Liu et al.[75] | PAM Cellulose | [BMIM][BF4] | n | -20.1 | 13.9 | 0.46 |
Sha et al.[84] | PAM SA | FeCl3 | n | -2.01 | 1.64 |
Ion conductor
In i-TE hydrogel, ion transport is primarily facilitated by salts and ILs, both of which play a significant role in determining TE performance. Salts dissociate in water, generating free ions that increase ion concentration, thus enhancing both ionic conductivity and Seebeck coefficient. However, their long-term stability can be compromised due to their evaporation and crystallization. Common salts such as LiCl, NaCl, and KCl contribute to TE performance by enabling thermally induced ion diffusion. In contrast, ILs consist of organic cations paired with either organic or inorganic anions, remaining in a liquid state over a broad temperature range due to their low vapor pressure and high thermal stability. ILs such as [BMIM][BF4] and [EMIM][TFSI] offer enhanced ion selectivity and tunability, increasing TE efficiency. Typically, salts are employed in p-type i-TE materials, where H3O+ or metal cations (e.g., Na+, Li+) serve as the primary charge carriers. Conversely, ILs are often incorporated into n-type systems, where mobile anions (e.g., BF4-, TFSI-) dominate charge transport. The selection and combination of these ionic conductors play a crucial role in determining overall i-TE performance, as they directly impact ion mobility, selectivity, and Seebeck coefficient.
Salt
Salts play a crucial role in enhancing ionic conductivity in i-TE hydrogels by dissociating into free ions upon dissolution in water. Commonly used salts include lithium salts (e.g., LiCl, LiBr), sodium salts (e.g., NaCl, NaBr), and potassium salts (e.g., KCl, KBr), all of which exhibit high solubility and provide a strong electrolyte environment within the hydrogel matrix. Once dissolved, cations and anions interact individually with water molecules through hydration, significantly affecting their mobility. For instance, Li+ ion, due to its small ionic radius and high charge density, forms a thick hydration shell that reduces its mobility. In contrast, K+ ion, with a larger radius and weaker hydration effects, exhibits higher diffusivity. These variations in ion mobility directly impact selective ion transport within the hydrogel, ultimately influencing its TE performance. By tailoring the choice of salts, hydrogel-based i-TE materials can be engineered to exhibit either p-type or n-type behavior, depending on the dominant ionic charge carrier.
In p-type i-TE, charge transport is primarily governed by cations such as Na+, K+, and H3O+. When incorporated into hydrogels, these cations diffuse along a thermal gradient, generating a positive Seebeck coefficient. The selection of salts plays a crucial role in determining the efficiency of thermally induced diffusion, as mobile cations preferentially migrate under heat stimulation. For instance, hydrogels containing NaCl exhibit p-type behavior due to the dominant role of Na+ ions in charge transport. Similarly, systems incorporating hydronium ions (H3O+) can achieve even higher TE performance, as protons exhibit exceptionally high mobility in aqueous environments.
Studies on PAM/LiCl-based hydrogels have demonstrated a Seebeck coefficient of 10.4 mV K-1, attributed to the efficient migration of Li+ ions. The small ionic radius and strong hydration of Li+ contribute to its rapid diffusion, optimizing thermodiffusion and enhancing TE performance through efficient cation mobility. However, He et al. showed that the ionic Seebeck coefficient in hydrogel systems is strongly influenced by the hydration structure and electrostatic interactions between ions and the polymer matrix
Figure 8. Schematic illustration of the thermal diffusion and ion migration, highlighting ionic TE properties influenced by ion type and size. (A) Schematic representation of ion thermal diffusion in a hydrogel, driven by the making and breaking of hydrogen bonds between ion and polymer chains in aqueous solution. Relationship between the Seebeck coefficient and the -ΔGHB values of (B) anions and (C) cations. (D) Viscosity and (E) Seebeck coefficient of ions in PVA hydrogel. Reproduced with permission[68]. Copyright 2022, American Chemical Society.
Ionic liquid
Ionic liquids (ILs) are important in i-TE hydrogels by influencing ion transport dynamics, ionic conductivity, and Seebeck coefficient. Unlike conventional salts, ILs consist of asymmetrically sized cations and anions, resulting in differences in ion mobility and charge separation efficiency. Their effectiveness in i-TE hydrogel is largely determined by cation-anion interactions, hydration effects, and polymer compatibility, all of which contribute to ion diffusivity and thermodiffusion asymmetry. The typical TE behavior of IL-based TE gels is p-type in anhydrous systems, where charge transport is dominated by cation migration. This phenomenon results from the size mismatch between cations and anions: larger cations, with lower frictional resistance, contribute more effectively to charge transport, while smaller anions experience higher environmental resistance. This imbalance results in a positive Seebeck coefficient. However, water absorption within hydrogels alters ion transport properties, as hydration reduces Coulombic interactions between ion pairs, thereby increasing overall ionic mobility. Additionally, the swelling of IL-based hydrogels selectively increases anion solvation, raising the hydrodynamic radius of anions while decreasing their mobility relative to cations. This asymmetry in solvation ultimately increases the Seebeck coefficient, indicating the importance of precise hydration control in i-TE hydrogels.
The effect of cation size and charge distribution on IL-based thermoelectrics has also been extensively studied. Horike et al. investigated imidazolium-based ILs with varying alkyl chain lengths and found that shorter alkyl chains (e.g., [EMIM]+) exhibited a higher Seebeck coefficient of 10.1 mV K-1, while longer alkyl chains (e.g., [DMIM]+ and [HMIM]+) led to lower values of 7.2 and 5.9 mV K-1, respectively
Figure 9. Molecular structures and TE properties of various ionic liquids, highlighting the effect of cation size and electrical charge. (A) Molecular structures of Rmim-Cl IL and poly(vinyl alcohol) (PVA). (B) TE properties of different ionic liquids, including Seebeck coefficient, ionic conductivity, and thermal conductivity. Reproduced with permission[80]. Copyright 2020, American Chemical Society; (C) Increase in -ΔV as a function of ΔT, representing the Seebeck coefficient by different ionic liquids (neat EMIM:TFSI, EMIM:TFSI/PVDF-HFP, and EMIM:TFSI/PVDF-HFP/PEG). Schematic representation of the primary ion conduction mechanism in ionic liquids, governed by (D) anions and (E) cations. Reproduced under the terms of the Creative Commons CC-BY license[87]. Copyright 2019, Springer Nature.
APPLICATION OF IONIC THERMOELECTRIC HYDROGEL
i-TE hydrogels operating in thermo-diffusive mode offer a promising approach for harvesting low-grade heat from the surrounding environment, making them particularly well-suited for wearable applications. Notably, the three-dimensional polymer framework of hydrogels ensures both mechanical flexibility and lightweight properties, enhancing their adaptability for thermal energy conversion in wearable and ambient applications. In this section, we discuss the potential of i-TE hydrogels functioning in thermo-diffusive mode in three key areas: capacitive energy storage systems, sensing platforms, and TE generators.
Hydrogel-based i-TE capacitors
A distinguishing feature of i-TE hydrogels driven by Soret effect is that the diffused ions do not directly migrate into the metal electrodes. Instead, these ions accumulate on the electrode surface, forming an electric double layer within the hydrogel, which induces a transient current. Consequently, i-TE hydrogels relying on the Soret effect are unsuitable for conventional TE generators that require a stable current output. i-TE hydrogels utilize temperature gradients to induce selective ion transport, enabling efficient charge separation and enhancing energy density. This unique mechanism has led to growing interest in developing self-charging ionic supercapacitors capable of efficiently converting low-grade heat into electrochemical energy. Hydrogel-based i-TE capacitors operate in four-stage cyclic process: (i) thermo-ionic charging; (ii) forward electronic working; (iii) thermo-ionic discharging by ion redistribution; and (iv) reverse electronic working. Ion carriers migrate to the electrode surface under a temperature gradient (∆T), forming an electric potential difference that enables the system to function as a capacitor. When the temperature gradient is removed, ions redistribute, and reconnecting the circuit completes the discharge cycle. This cyclic process enables continuous thermal energy harvesting and charge storage, making hydrogel-based i-TE capacitors highly suitable for soft electronic devices.
Fu et al. developed highly stretchable, resilient, adhesive, and self-healing ionic hydrogels for TE applications[88]. These hydrogels, composed of a physically cross-linked network of polyacrylic acid (PAA) and polyethylene glycol (PEO) doped with sodium chloride (NaCl), exhibited remarkable mechanical properties, including a breaking stress exceeding 1.3 MPa, stretchability above 1,100%, and toughness reaching 7.34 MJ m-3. Furthermore, the hydrogels achieved a Seebeck coefficient of 3.26 mV K-1 with a low thermal conductivity of 0.321 W m-1K-1. PAA-PEO-3MNaCl ionic hydrogels experience a four-stage TE cycle, enabling continuous charge generation and storage. This system responds sensitively to small temperature gradients and maintains performance under varying electrical loads, demonstrating its potential for low-grade heat utilization [Figure 10A-D].
Figure 10. Ionic thermoelectric supercapacitor (ITESC). (A) Schematic illustration of the operation mechanism at the four stages of an ITESC with the PAA-PEO-3MNaCl ionic hydrogels; (B) Thermo-ionic charging and electronic discharging four-stages curve; (C) Voltage profiles of the external loads with different resistances; (D) Plot of the total charging and discharging energy versus the resistance of the external load. Reproduced with permission[88]. Copyright 2023, Wiley-VCH GmbH; (E) Thermal-ionic charging and electronic discharging four-stages curve of the PAM/CMC-2LiCl ionic hydrogel; (F) Schematic diagram of an ITESC consisting of 5 legs; (G) Thermal voltage depending on the number of legs. Reproduced with permission[35]. Copyright 2024, Elsevier Ltd.; (H) Schematic illustration of i-TE hydrogel electrolyte with corresponding voltage and temperature profiles; (I) Current density and power density versus output voltage. Reproduced with permission[36]. Copyright 2025, Royal Society of Chemistry.
Qian et al. introduced a highly stretchable, low-hysteresis, and antifreeze hydrogel designed for low-grade thermal energy harvesting in i-TE supercapacitors[35]. The hydrogel, formulated with a dual network structure of PAM and sodium carboxymethyl cellulose (CMC) with lithium chloride (LiCl) as the conductive component, demonstrated outstanding mechanical properties. The presence of LiCl enhanced the hydrogel’s ionic conductivity to 36.51 mS cm-1 while simultaneously providing freeze resistance and the ability to absorb moisture for self-regeneration upon drying. The hydrogel-based ITESC device exhibited Soret-effect-driven voltage generation, with output scaling nearly linearly with the number of TE legs. A voltage of 0.182 V was achieved using five units under a 12 K temperature gradient [Figure 10E-G]. These results confirm that stacking multiple hydrogel units effectively enhances the thermo-voltage output.
Li et al. presented a novel approach by utilizing an interpenetrating polymer network composed of sodium PSS-modified PVA (PVA-PSS), which effectively enhances anion diffusion while restricting cation movement[36]. This ionic transport asymmetry enhances thermally driven charge separation and improves energy conversion efficiency. Compared to PVA-H2O-KCl (PHK, 5.5 mW m-2) and PVA-H2O/DMSO-KCl (PDK, 27.2 mW m-2), the PVA-H2O/DMSO-PSS-KCl (PDSK) hydrogel exhibited superior performance, primarily due to enhanced anion diffusion and stronger electrostatic interactions within the polymer network [Figure 10H and I]. As research continues to address existing challenges in TE efficiency, mechanical durability, and environmental adaptability, the future of self-charging ionic supercapacitors appears increasingly promising for sustainable energy applications.
Hydrogel-based i-TE sensors
Hydrogels, known for their exceptional flexibility, tissue-like softness, adhesiveness, and ionic conductivity, have been widely used in wearable electronics, biomedical monitoring, and human-machine interfaces. Significantly, the main advantages of i-TE sensors for human monitoring applications include high flexibility, sensitivity, and durability under long-term cyclic mechanical loads, ensuring practical usability through a high Seebeck coefficient.
Chen et al. introduced a super-stretchable, high-performance i-TE hydrogel based on carboxylated BC (TOBC), designed for self-powered sensing applications[37]. The hydrogel incorporates LiTFSI as an ion provider, enhancing selective ion transport and yielding a high Seebeck coefficient of 11.53 mV K-1. This material offered exceptional mechanical properties, with tensile deformation up to 3,100% and a stress value of 0.85 MPa, facilitated by hydrogen bonding within the PAM network and borate ester interactions in TOBC. The TOBC-based i-TE hydrogel demonstrated excellent mechanical durability and real-time applicability for wearable sensing. When integrated with a boost converter and an Arduino-based circuit, it enabled wireless signal transmission for real-time monitoring of physiological movements. Applied to the human palm, the device accurately distinguished between bending and stretching motions, with signals successfully transmitted to a mobile interface [Figure 11A-H].
Figure 11. Ionic thermoelectric sensors (1). (A) The voltage-time and current-time curves of the hydrogel being repeatedly bent at 60°. (B) Photos of bend deformation states of i-TE hydrogel, (C) The variation and retention rate of the Seebeck coefficient and conductivity after 100 bending cycles, (D) The voltage-time and current-time curves of the hydrogel being repeatedly stretched at 100 %, (E) Photos of strain deformation states of i-TE hydrogel, (F) The variation and retention rate of the Seebeck coefficient and conductivity after 100 strain cycles, (G) Schematic diagram of the wireless signal transmission system, (H) The changes in the bending and stretching of the palm are displayed on the mobile phone in real time through wireless technology. Reproduced with permission[37]. Copyright 2023, Elsevier Ltd.
Han et al. developed ultrasensitive flexible thermal sensor arrays utilizing high-thermopower i-TE hydrogels for enhanced thermal sensing[40]. A smart glove integrated with PQ-10/NaOH-based i-TE hydrogel sensor arrays demonstrated real-time thermal sensing capabilities for wearable electronics. Each finger was equipped with flexible sensors patterned onto a flexible printed circuit board, allowing the device to detect and distinguish temperature variations upon contact with diverse objects. The hydrogel generated distinct TE responses - negative voltages for warm surfaces and positive voltages for cold - while maintaining stability under mechanical deformation. This responsive and flexible configuration highlights the potential of i-TE materials for tactile thermal sensing in intelligent wearables [Figure 12A-H].
Figure 12. Ionic thermoelectric sensors (2). (A) Photographs of a smart glove integrated with multiple thermal sensor arrays. (B) Photograph of the flexible thermal sensor array attached onto each finger. Demonstration of the thermal sensation function of the smart glove when in contact with (C, F) a hand warmer, (D, G) an ice-cold soda can, and (E, H) a human hand. Reproduced under the terms of the Creative Commons CC-BY license[40]. Copyright 2023, Wiley-VCH GmbH.
Hydrogel-based i-TE generators
In contrast to e-TE generators, the i-TE generators (ITEG) based on Soret effect exhibit capacitive behavior, as neither ions nor electrons can transport across the electrode-electrolyte interface. Due to the unique characteristics of ITEGs, they are inherently required to generate current through an indirect method[52]. The ITEG required a prior voltage build-up step (charging). When the external circuit is connected after voltage build-up step, a transient current is generated in the external circuit (discharging). This power supply gradually decays, depending on the external load resistance and the capacitive voltage of ITEG. This behavior is principally analogous to the charging process of i-TE capacitors.
Fu et al. introduced a thermosensitive ionic hydrogel with an integrated TE energy harvesting capability, expanding its applications to adaptive thermal management and energy-efficient building technologies
Figure 13. Ionic thermoelectric generators (ITEG). (A) i-TE characterization of the PAA-PEO-SDC ionic hydrogels: output power density measured under different external loads. Reproduced with permission[89]. Copyright 2024, Wiley-VCH GmbH; (B) ITEGs with PAM-LiCl-based double-network hydrogel. Reproduced with permission[90]. Copyright 2022, American Chemical Society.
CHALLENGES AND PERSPECTIVE
Hydrogel-based i-TE materials have emerged as promising candidates for energy harvesting applications due to their high ionic conductivity, mechanical flexibility, and biocompatibility. These materials efficiently convert low-grade heat into electrical energy through ion migration driven by a thermal gradient, achieving significantly higher Seebeck coefficients compared to conventional e-TE materials. Despite their potential, several critical challenges must be addressed before hydrogel-based i-TE systems can be practically applied. Notably, the limitations of hydrogel-based i-TE materials primarily arise from their inherent characteristics such as dehydration, mechanical degradation, and instability under fluctuating environmental conditions.
The main challenge is maintaining long-term water stability. Dehydration significantly compromises ionic conductivity, TE performance, and mechanical integrity, leading to rapid material degradation[35]. To mitigate this issue, strategies such as the introduction of encapsulation layers[40], integration of hygroscopic agents[91], and the design of hierarchical porous structures[92] have been explored to retain moisture. Additionally, developing multifunctional coatings that offer both waterproofing and mechanical durability shows promise. Nonetheless, achieving long-term hydration while preserving mechanical flexibility remains a fundamental challenge, necessitating innovative material designs and advanced engineering solutions. Another major limitation is the inherently low ionic conductivity of hydrogel-based i-TE materials, which restricts their energy conversion efficiency. To address this issue, hybrid hydrogels incorporating conductive materials such as carbon nanotubes, graphene, or conductive polymers have been developed[32]. These hybrid structures improve the electrical conductivity without compromising the intrinsic flexibility and ionic mobility of the hydrogel matrix, making them more viable for real-world applications. Mechanical durability under repeated thermal and mechanical stress, particularly in wearable or dynamic environments, is also a significant concern. Due to their soft and water-rich nature, hydrogels are prone to mechanical damage under cyclic deformation. Strategies to enhance mechanical robustness include the design of double-network hydrogels, incorporation of reinforcing composites, and the use of self-healing materials. Additionally, embedding nanomaterials such as carbon nanotubes or graphene can further reinforce the hydrogel matrix, improving both mechanical strength and electrical performance. Maintaining consistent TE performance across a wide temperature range presents another challenge. High temperatures accelerate water evaporation, while low temperatures may induce freezing or rigidity, both of which hinder stable performance. Solutions such as integrating low-volatile ILs or developing freeze-resistant hydrogels have shown promise in expanding operational temperature windows. Moreover, a fundamental distinction between i-TE and e-TE materials lies in their environmental stability and TE performance. As illustrated in Figure 14, while i-TE hydrogels offer high flexibility and biocompatibility, their performance is significantly influenced by external factors such as humidity and temperature. In contrast, e-TE materials typically maintain more stable operation under varying environmental conditions. As a result, overcoming the current limitations of hydrogel-based i-TE systems requires the development of advanced polymer matrices with improved water retention, mechanical robustness, and electrical conductivity. Future research should also focus on hybrid systems that integrate conductive components to mitigate the intrinsic drawbacks of pure hydrogels. By deepening our understanding of ion transport mechanisms and optimizing polymer-ion interactions, hydrogel-based i-TE materials can be transformed into practical and efficient energy harvesting solutions for applications in wearable electronics, biomedical devices, and sustainable power systems.
Figure 14. Comparative performance diagram of ionic thermoelectrics and electronic thermoelectrics. (A) Comparison of TE performance indicators: ZT, Seebeck coefficient, thermal conductivity, power factor, and electrical/ionic conductivity (clockwise); (B) Mechanical properties and stability against external environmental factors.
CONCLUSION
Hydrogel-based i-TE materials have demonstrated a remarkable potential for low-grade heat harvesting by utilizing ion migration under a thermal gradient. Compared to conventional e-TE materials that rely on electron transport, i-TE materials use the Soret effect to drive selective ion diffusion, achieving significantly higher Seebeck coefficients. This unique mechanism makes i-TE hydrogels highly attractive for applications in wearable electronics, biomedical devices, and sustainable power generation. A key factor that distinguishes i-TE materials from traditional e-TE materials is the complex interplay of ion-matrix-solvent interactions, which fundamentally governs ion mobility and TE performance. The nature and strength of ion interactions with the polymer network, hydration shells, and other ions critically influence charge separation and ionic conductivity. For instance, the difference in hydration energy between cations and anions directly affects selective ion transport and the resulting thermo-voltage. Incorporating functional groups that interact strongly with specific ions can enhance ion selectivity, allowing for tailored ion migration pathways. Additionally, balancing hydration stability and ionic mobility is crucial for maintaining consistent TE performance, especially under varying environmental conditions. Despite significant advancements, challenges remain before hydrogel-based i-TE materials can be fully utilized in practical applications. One major challenge is maintaining long-term water retention, as dehydration can significantly degrade ionic conductivity and TE efficiency. Enhancing mechanical durability under repeated stress is also essential to ensure reliability in daily life. Furthermore, optimizing the balance between ionic conductivity and thermal stability remains critical to achieving high energy conversion efficiency. We hope that this review establishes a comprehensive theoretical framework for i-TE materials, providing deeper insights into their transport mechanisms and guiding the design of high-performance i-TE hydrogels. By advancing our understanding of ion-matrix-solvent interactions and optimizing material compositions, future developments can drive the development of practical and efficient energy harvesting systems.
DECLARATIONS
Authors’ contributions
Proposed the topic of this review: Kim, H.
Wrote the manuscript, designed and drew the Figures: Choi, Y.; Kang, B. C.
Discussed and revised the manuscript: Kim, J. Y.; Kang, B. C.; Kim, H.
Availability of data and materials
Not applicable.
Financial support and sponsorship
This work was supported by the National Research Foundation of Korea (NRF) (RS-2024-00452255 and RS-2024000449743).
Conflicts of interest
All authors declared that there are no conflicts of interest.
Ethical approval and consent to participate
Not applicable.
Consent for publication
Not applicable.
Copyright
© The Author(s) 2025.
REFERENCES
1. He, W.; Zhang, G.; Zhang, X.; Ji, J.; Li, G.; Zhao, X. Recent development and application of thermoelectric generator and cooler. Appl. Energy. 2015, 143, 1-25.
2. Zhang, A.; Pang, D.; Wang, B.; Wang, J. Dynamic responses of wearable thermoelectric generators used for skin waste heat harvesting. Energy 2023, 262, 125621.
3. Li, C.; Jiang, F.; Liu, C.; Liu, P.; Xu, J. Present and future thermoelectric materials toward wearable energy harvesting. Appl. Mater. Today. 2019, 15, 543-57.
4. Lu, N.; Li, L.; Liu, M. A review of carrier thermoelectric-transport theory in organic semiconductors. Phys. Chem. Chem. Phys. 2016, 18, 19503-25.
5. Tang, W.; Qian, W.; Jia, S.; et al. BiCuSeO based thermoelectric materials: innovations and challenges. Mater. Today. Phys. 2023, 35, 101104.
6. Wang, Y.; Yang, L.; Shi, X. L.; et al. Flexible thermoelectric materials and generators: challenges and innovations. Adv. Mater. 2019, 31, e1807916.
7. Feng, J.; Li, J.; Liu, R. Low-temperature thermoelectric materials and applications. Nano. Energy. 2024, 126, 109651.
8. Zhao, X.; Chen, Z.; Zhuo, H.; et al. Thermoelectric generator based on anisotropic wood aerogel for low-grade heat energy harvesting. J. Mater. Sci. Technol. 2022, 120, 150-8.
9. Bu, Z.; Zhang, X.; Hu, Y.; et al. A record thermoelectric efficiency in tellurium-free modules for low-grade waste heat recovery. Nat. Commun. 2022, 13, 237.
10. Ang, A. K. R.; Yamazaki, I.; Hirata, K.; Singh, S.; Matsunami, M.; Takeuchi, T. Development of Cu2Se/Ag2(S,Se)-based monolithic thermoelectric generators for low-grade waste heat energy harvesting. ACS. Appl. Mater. Interfaces. 2023, 15, 46962-70.
11. Xu, C.; Liang, Z.; Ren, W.; Song, S.; Zhang, F.; Ren, Z. Realizing high energy conversion efficiency in a novel segmented-
12. Liu, Z.; Gao, W.; Oshima, H.; Nagase, K.; Lee, C. H.; Mori, T. Maximizing the performance of n-type Mg3Bi2 based materials for room-temperature power generation and thermoelectric cooling. Nat. Commun. 2022, 13, 1120.
13. Li, W.; Poudel, B.; Kishore, R. A.; et al. Toward high conversion efficiency of thermoelectric modules through synergistical optimization of layered materials. Adv. Mater. 2023, 35, e2210407.
14. Soleimani, Z.; Zoras, S.; Ceranic, B.; Cui, Y.; Shahzad, S. A comprehensive review on the output voltage/power of wearable thermoelectric generators concerning their geometry and thermoelectric materials. Nano. Energy. 2021, 89, 106325.
15. Lee, B.; Cho, H.; Park, K. T.; et al. High-performance compliant thermoelectric generators with magnetically self-assembled soft heat conductors for self-powered wearable electronics. Nat. Commun. 2020, 11, 5948.
16. Jeong, Y. J.; Jung, J.; Suh, E. H.; Yun, D.; Oh, J. G.; Jang, J. Self-healable and stretchable organic thermoelectric materials: electrically percolated polymer nanowires embedded in thermoplastic elastomer matrix. Adv. Funct. Mater. 2020, 30, 1905809.
17. Akbar, Z. A.; Malik, Y. T.; Kim, D. H.; Cho, S.; Jang, S. Y.; Jeon, J. W. Self-healable and stretchable ionic-liquid-based thermoelectric composites with high ionic Seebeck coefficient. Small 2022, 18, e2106937.
18. He, H.; Ouyang, J. Enhancements in the mechanical stretchability and thermoelectric properties of PEDOT:PSS for flexible electronics applications. Acc. Mater. Res. 2020, 1, 146-57.
19. Zhao, D.; Würger, A.; Crispin, X. Ionic thermoelectric materials and devices. J. Energy. Chem. 2021, 61, 88-103.
20. Jia, S.; Qian, W.; Yu, P.; et al. Ionic thermoelectric materials: innovations and challenges. Mater. Today. Phys. 2024, 42, 101375.
21. Fu, M.; Sun, Z.; Yuan, Y.; Yue, K. Ionic thermoelectric materials based on the thermodiffusion effect: mechanism, advancements, and applications. Macro. Chem. Phys. 2025, 226, 2400358.
22. Lee, S. W.; Yang, Y.; Lee, H. W.; et al. An electrochemical system for efficiently harvesting low-grade heat energy. Nat. Commun. 2014, 5, 3942.
23. Mentor, J. J.; Torres, R.; Hallinan, D. T. The soret effect in dry polymer electrolyte. Mol. Syst. Des. Eng. 2020, 5, 856-63.
24. Li, M.; Hong, M.; Dargusch, M.; Zou, J.; Chen, Z. High-efficiency thermocells driven by thermo-electrochemical processes. Trends. Chem. 2021, 3, 561-74.
25. Zhao, D.; Wang, H.; Khan, Z. U.; et al. Ionic thermoelectric supercapacitors. Energy. Environ. Sci. 2016, 9, 1450-7.
26. Fang, Y.; Cheng, H.; He, H.; et al. Stretchable and transparent ionogels with high thermoelectric properties. Adv. Funct. Mater. 2020, 30, 2004699.
27. Cheng, H.; Le, Q.; Liu, Z.; Qian, Q.; Zhao, Y.; Ouyang, J. Ionic thermoelectrics: principles, materials and applications. J. Mater. Chem. C. 2022, 10, 433-50.
28. Cheng, H.; He, X.; Fan, Z.; Ouyang, J. Flexible quasi-solid state ionogels with remarkable Seebeck coefficient and high thermoelectric properties. Adv. Energy. Mater. 2019, 9, 1901085.
29. Chen, B.; Chen, Q.; Xiao, S.; Feng, J.; Zhang, X.; Wang, T. Giant negative thermopower of ionic hydrogel by synergistic coordination and hydration interactions. Sci. Adv. 2021, 7, eabi7233.
30. Li, T.; Zhang, X.; Lacey, S. D.; et al. Cellulose ionic conductors with high differential thermal voltage for low-grade heat harvesting. Nat. Mater. 2019, 18, 608-13.
31. Ke, J.; Zhao, X.; Yang, J.; et al. Enhanced ion-selective diffusion achieved by supramolecular interaction for high thermovoltage and thermal stability. Energy. Environ. Mater. 2024, 7, e12562.
32. Wang, Z.; Li, N.; Zhang, Z.; Cui, X.; Zhang, H. Hydrogel-based energy harvesters and self-powered sensors for wearable applications. Nanoenergy. Adv. 2023, 3, 315-42.
33. Zhang, C.; Shi, X.; Liu, Q.; Chen, Z. Hydrogel-based functional materials for thermoelectric applications: progress and perspectives. Adv. Funct. Mater. 2024, 34, 2410127.
34. Yu, M.; Li, H.; Li, Y.; et al. Ionic thermoelectric gels and devices: progress, opportunities, and challenges. EnergyChem 2024, 6, 100123.
35. Qian, W.; Jia, S.; Yu, P.; et al. Highly stretchable, low-hysteresis, and antifreeze hydrogel for low-grade thermal energy harvesting in ionic thermoelectric supercapacitors. Mater. Today. Phys. 2024, 49, 101589.
36. Li, S.; Xu, Y.; Li, Z.; Zhang, S.; Dou, H.; Zhang, X. An n-type ionic thermoelectric hydrogel with confined cation diffusion for boosted low-grade heat harvesting. J. Mater. Chem. A. 2025, 13, 3913-21.
37. Chen, L.; Lou, J.; Rong, X.; et al. Super-stretching and high-performance ionic thermoelectric hydrogels based on carboxylated bacterial cellulose coordination for self-powered sensors. Carbohydr. Polym. 2023, 321, 121310.
38. Dai, Y.; Wang, H.; Qi, K.; et al. Electrode-dependent thermoelectric effect in ionic hydrogel fiber for self-powered sensing and low-grade heat harvesting. Chem. Eng. J. 2024, 497, 154970.
39. Zhang, Y.; Dai, Y.; Xia, F.; Zhang, X. Gelatin/polyacrylamide ionic conductive hydrogel with skin temperature-triggered adhesion for human motion sensing and body heat harvesting. Nano. Energy. 2022, 104, 107977.
40. Han, Y.; Wei, H.; Du, Y.; et al. Ultrasensitive flexible thermal sensor arrays based on high-thermopower ionic thermoelectric hydrogel. Adv. Sci. 2023, 10, e2302685.
41. Lee, C. Y.; Hong, S. H.; Liu, C. L. Recent progress in polymer gel-based ionic thermoelectric devices: materials, methods, and perspectives. Macromol. Rapid. Commun. 2025, 46, e2400837.
42. Son, C. Y.; Wang, Z. G. Ion transport in small-molecule and polymer electrolytes. J. Chem. Phys. 2020, 153, 100903.
43. Wu, Z.; Wang, B.; Li, J.; et al. Advanced bacterial cellulose ionic conductors with gigantic thermopower for low-grade heat harvesting. Nano. Lett. 2022, 22, 8152-60.
44. Madduma-Bandarage, U. S. K.; Madihally, S. V. Synthetic hydrogels: synthesis, novel trends, and applications. J. Appl. Polym. Sci. 2021, 138, 50376.
45. Hsiao, Y. C.; Lee, L. C.; Lin, Y. T.; et al. Stretchable polyvinyl alcohol and sodium alginate double network ionic hydrogels for low-grade heat harvesting with ultrahigh thermopower. Mater. Today. Energy. 2023, 37, 101383.
46. Yossef, M.; Baniasadi, H.; Kallio, T.; Perry, M.; Puttonen, J. Ionic thermoelectricity of salt-free PVA-hydrogel. Appl. Mater. Today. 2024, 38, 102240.
47. Yang, X.; Tian, Y.; Wu, B.; et al. High-performance ionic thermoelectric supercapacitor for integrated energy conversion-storage. Energy. Environ. Mater. 2022, 5, 954-61.
48. Wang, Y.; Liu, X.; Li, S.; et al. Transparent, healable elastomers with high mechanical strength and elasticity derived from hydrogen-bonded polymer complexes. ACS. Appl. Mater. Interfaces. 2017, 9, 29120-9.
49. Li, Q.; Han, C. G.; Wang, S.; et al. Anionic entanglement-induced giant thermopower in ionic thermoelectric material Gelatin-CF3SO3K-CH3SO3K. eScience 2023, 3, 100169.
50. Chen, B.; Feng, J.; Chen, Q.; et al. Specific behavior of transition metal chloride complexes for achieving giant ionic thermoelectric properties. NPJ. Flex. Electron. 2022, 6, 213.
51. Sui, X.; Guo, H.; Cai, C.; et al. Ionic conductive hydrogels with long-lasting antifreezing, water retention and self-regeneration abilities. Chem. Eng. J. 2021, 419, 129478.
52. Sun, S.; Li, M.; Shi, X.; Chen, Z. Advances in ionic thermoelectrics: from materials to devices. Adv. Energy. Mater. 2023, 13, 2203692.
53. Kishore, R. A.; Nozariasbmarz, A.; Poudel, B.; Sanghadasa, M.; Priya, S. Ultra-high performance wearable thermoelectric coolers with less materials. Nat. Commun. 2019, 10, 1765.
54. Wang, H.; Zhao, D.; Khan, Z. U.; et al. Ionic thermoelectric figure of merit for charging of supercapacitors. Adv. Electron. Mater. 2017, 3, 1700013.
55. Lan, J. L.; Ma, W.; Deng, C.; Ren, G. K.; Lin, Y. H.; Yang, X. High thermoelectric performance of Bi1-xKx CuSeO prepared by combustion synthesis. J. Mater. Sci. 2017, 52, 11569-79.
56. Massetti, M.; Jiao, F.; Ferguson, A. J.; et al. Unconventional thermoelectric materials for energy harvesting and sensing applications. Chem. Rev. 2021, 121, 12465-547.
57. Yang, L.; Chen, Z.; Dargusch, M. S.; Zou, J. High performance thermoelectric materials: progress and their applications. Adv. Energy. Mater. 2018, 8, 1701797.
58. de Groot, S. R.; Mazur, P.; Choi, S. Non-equilibrium thermodynamics. Phys. Today. 1963, 16, 70-1.
61. Liu, J.; Zeng, W.; Tao, X. Gigantic effect due to phase transition on thermoelectric properties of ionic sol-gel materials. Adv. Funct. Mater. 2022, 32, 2208286.
63. Paulsen, B. D.; Tybrandt, K.; Stavrinidou, E.; Rivnay, J. Organic mixed ionic-electronic conductors. Nat. Mater. 2020, 19, 13-26.
64. Jia, S.; Qian, W.; Yu, P.; et al. Realization of hydrogel electrolytes with high thermoelectric properties: utilization of the hofmeister effect. ACS. Appl. Mater. Interfaces. 2024, 16, 69519-28.
65. Cheng, H.; Wang, Z.; Guo, Z.; et al. Cellulose-based thermoelectric composites: a review on mechanism, strategies and applications. Int. J. Biol. Macromol. 2024, 275, 132908.
66. Liu, Y.; Zhang, Q.; Odunmbaku, G. O.; et al. Solvent effect on the Seebeck coefficient of Fe2+/Fe3+ hydrogel thermogalvanic cells. J. Mater. Chem. A. 2022, 10, 19690-8.
67. Zhou, Y.; Dong, Z.; He, Y.; et al. Multi-ionic hydrogel with outstanding heat-to-electrical performance for low-grade heat harvesting. Chem. Asian. J. 2022, 17, e202200850.
68. He, Y.; Zhang, Q.; Cheng, H.; et al. Role of ions in hydrogels with an ionic Seebeck coefficient of 52.9 MV K-1. J. Phys. Chem. Lett. 2022, 13, 4621-7.
69. Cheng, H.; Ouyang, J. Soret effect of ionic liquid gels for thermoelectric conversion. J. Phys. Chem. Lett. 2022, 13, 10830-42.
70. Muddasar, M.; Menéndez, N.; Quero, Á.; et al. Highly-efficient sustainable ionic thermoelectric materials using lignin-derived hydrogels. Adv. Compos. Hybrid. Mater. 2024, 7, 863.
71. Zhou, Y.; Yang, L.; Xu, J.; Wei, Z.; Ma, X.; Yuan, B. A bio-based alginate hydrogel with considerable thermoelectric performance, mechanical strength and flame retardancy for ultra-fast and sustained early fire-alarm system. Int. J. Biol. Macromol. 2025, 300, 140324.
72. Zhou, Z.; Wan, Y.; Zi, J.; et al. Flexible hydrogel with a coupling enhanced thermoelectric effect for low-grade heat harvest. Mater. Today. Sustain. 2023, 21, 100293.
73. Ajdary, R.; Tardy, B. L.; Mattos, B. D.; Bai, L.; Rojas, O. J. Plant nanomaterials and inspiration from nature: water interactions and hierarchically structured hydrogels. Adv. Mater. 2021, 33, e2001085.
74. Hu, Y.; Chen, M.; Qin, C.; Zhang, J.; Lu, A. Cellulose ionic conductor with tunable Seebeck coefficient for low-grade heat harvesting. Carbohydr. Polym. 2022, 292, 119650.
75. Liu, K. Y.; Hu, Y. P.; Yu, W.; Meng, C. Z.; Guo, S. J.; Li, G. X. Cellulose nanofibrils enhanced thermoelectric hydrogel with high negative Seebeck coefficient. Mater. Lett. 2024, 372, 137038.
76. Cheng, X.; Hu, Y.; Chen, P.; Qi, H.; Lu, A. Regulation of thermal migration channel in cellulose hydrogel to enhance thermopower. Chem. Eng. J. 2024, 498, 155161.
77. Chen, L.; Rong, X.; Liu, Z.; et al. Negative thermopower anisotropic ionic thermoelectric hydrogels based on synergistic coordination and hydration for low-grade heat harvesting. Chem. Eng. J. 2024, 481, 148797.
78. Liang, X.; Zhong, H. J.; Ding, H.; et al. Polyvinyl alcohol (pva)-based hydrogels: recent progress in fabrication, properties, and multifunctional applications. Polymers 2024, 16, 2755.
79. Chen, Q.; Chen, B.; Xiao, S.; et al. Giant thermopower of hydrogen ion enhanced by a strong hydrogen bond system. ACS. Appl. Mater. Interfaces. 2022, 14, 19304-14.
80. Horike, S.; Wei, Q.; Kirihara, K.; et al. Outstanding electrode-dependent Seebeck coefficients in ionic hydrogels for thermally chargeable supercapacitor near room temperature. ACS. Appl. Mater. Interfaces. 2020, 12, 43674-83.
81. Hu, Q.; Li, H.; Chen, X.; et al. Strong tough ionic organohydrogels with negative-thermopower via the synergy of coordination interaction and hofmeister effect. Adv. Funct. Mater. 2024, 34, 2406968.
82. Sennakesavan, G.; Mostakhdemin, M.; Dkhar, L.; Seyfoddin, A.; Fatihhi, S. Acrylic acid/acrylamide based hydrogels and its properties - a review. Polym. Degrad. Stab. 2020, 180, 109308.
83. Jiang, C.; Lai, X.; Wu, Z.; et al. A high-thermopower ionic hydrogel for intelligent fire protection. J. Mater. Chem. A. 2022, 10, 21368-78.
84. Sha, W.; Wang, Y.; Xiao, M.; et al. Conductive ionic thermoelectric hydrogel with negative Seebeck coefficient, self-healing and highly sensitive to temperature for photothermoelectric conversion and non-contact sensing device. Chem. Eng. J. 2024, 501, 157823.
85. Chen, J.; Zhang, L.; Tu, Y.; et al. Wearable self-powered human motion sensors based on highly stretchable quasi-solid state hydrogel. Nano. Energy. 2021, 88, 106272.
86. Liu, C.; Li, Q.; Wang, S.; Liu, W.; Fang, N. X.; Feng, S. Ion regulation in double-network hydrogel module with ultrahigh thermopower for low-grade heat harvesting. Nano. Energy. 2022, 92, 106738.
87. Zhao, D.; Martinelli, A.; Willfahrt, A.; et al. Polymer gels with tunable ionic Seebeck coefficient for ultra-sensitive printed thermopiles. Nat. Commun. 2019, 10, 1093.
88. Fu, M.; Sun, Z.; Liu, X.; et al. Highly stretchable, resilient, adhesive, and self-healing ionic hydrogels for thermoelectric application. Adv. Funct. Mater. 2023, 33, 2306086.
89. Fu, M.; Yuan, Y.; Liu, X.; et al. A thermosensitive ionic hydrogel for thermotropic smart windows with integrated thermoelectric energy harvesting capability. Adv. Funct. Mater. 2025, 35, 2412081.
90. Chen, J.; Shi, C.; Wu, L.; et al. Environmentally tolerant ionic hydrogel with high power density for low-grade heat harvesting. ACS. Appl. Mater. Interfaces. 2022, 14, 34714-21.
91. Wu, H.; Chen, G.; Xie, S.; Xiang, K.; Fan, Y.; Guo, Z. Waste-heat harvesting using a thermoelectric generator coupled with a hygroscopic hydrogel for use in the energy industry. J. Mater. Chem. C. 2025, 13, 1801-11.
Cite This Article

How to Cite
Download Citation
Export Citation File:
Type of Import
Tips on Downloading Citation
Citation Manager File Format
Type of Import
Direct Import: When the Direct Import option is selected (the default state), a dialogue box will give you the option to Save or Open the downloaded citation data. Choosing Open will either launch your citation manager or give you a choice of applications with which to use the metadata. The Save option saves the file locally for later use.
Indirect Import: When the Indirect Import option is selected, the metadata is displayed and may be copied and pasted as needed.
About This Article
Special Issue
Copyright
Data & Comments
Data
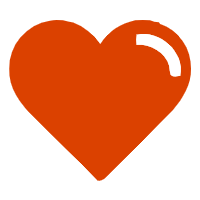
Comments
Comments must be written in English. Spam, offensive content, impersonation, and private information will not be permitted. If any comment is reported and identified as inappropriate content by OAE staff, the comment will be removed without notice. If you have any queries or need any help, please contact us at support@oaepublish.com.