Mechanosensitive apoptosis in Alzheimer′s disease
Abstract
The incidence of neurodegenerative diseases is increasing exponentially, with Alzheimer’s disease (AD) being a notorious example. AD is a progressive, aging-related, multifactorial disease, and no uniform theory exists regarding its etiology. One hypothesis implicates defects in the organism’s mechanosensing and mechanotransduction mechanisms. The deleterious effects of mechanical stress in neurodegeneration have been proven through multiple studies and observations, but the pathways linking mechanical inputs to disease progression remain unclear. It has been proposed that the nervous tissue primarily responds to mechanical stress sources through astrocytes and microglia, with the activation of Piezo1 and TRPV4 ion channels. An area of current research is examining the theory that mechanotransduction might be involved in elevated oxidative stress and dysregulated apoptotic pathways. We have summarized the current understanding of the apoptotic pathways related to AD. Our review has indicated that the relationship between the crucial mechanosensing Hippo pathway (MST1 in humans) and TRPV4 and KCNN4 is worth following up in future studies. We noted that G3BP1 and G3BP2 in FlnA-mediated mechanosensing are linked to ceramide production, along with their interaction with the stress granules - a key element for the onset of apoptosis, which are assembled, among others, in response to oxidative stress. This review sheds light on how the response to mechanical input disrupts apoptotic pathways and what the implications in AD progression and also identifies potential targets for future research focus.
Keywords
INTRODUCTION
Alzheimer’s disease (AD) is the curse of the age group of 65 and above, as scientists predict a dementia-related pandemic. As of 2024, an estimated 6.9 million people in the United States alone are living with AD, making it the 5th leading cause of death[1]. AD affects populations worldwide, imposing substantial social and financial burdens[2]. AD symptoms typically manifest long after the onset of the underlying pathology[3], which is why early diagnosis remains a major focus of current research efforts[4].
AD belongs to a group of diseases originating from abnormal protein aggregates, and current perspectives on AD highlight two primary culprits: β-amyloid plaques outside neuron bodies and hyperphosphorylated tau within them[5]. While the presence and pathological significance of both are well established, ongoing debates center on which is the primary factor and how they interact with each other[6].
The etiology of AD appears to be multifactorial. Recent discoveries suggest a genetic component to its heritability[7], while others advocate the role of inflammation triggered by infectious agents[8] and alterations in gut microbiota[9]. The underlying factors lead to excessive inflammation, oxidative stress, DNA damage, synaptic loss, and neuronal apoptosis[10-12]. Additionally, emerging evidence points to aberrant mechanosensitive apoptosis (MSA) as a potential contributing mechanism[13]. A summary of the gist findings reviewed is offered in Table 1.
Summary of mechanosensitive properties of nervous system cells
Cell type | Summary |
Astrocytes![]() Created in BioRender. S, G. (2025) https://BioRender.com/ab7uib8 |
Linked to heart and metabolism through mechanosensing. Proliferate in stiff environments and increase the stiffness of glial cells. In optic nerves, mechanical stimulation activates Piezo1 signaling, potentially mediated by oxidative stress. Perform phagocytic functions by clearing cellular debris (dead cells, etc.). Piezo1 reduction in astrocytes decreases brain and neuronal volume, impairing brain function, while Piezo1 activation has the opposite effect. Die in epileptic seizures as a result of inflammasome cascade |
Microglia![]() Created in BioRender. S, G. (2025) https://BioRender.com/ab7uib8 |
Key mechanosensitive cells involved in inflammation, where the Hippo pathway plays a protective role. Upon sensing stiff amyloid-β aggregates, microglia become activated, enabling clearance but also causing inflammation and neuron damage. They also respond to concussive trauma in the long term and to ultrasound stimulation with increased apoptosis. Mechanical input elevates inflammasome activity via TRPV4, and TRPV4 agonists can reduce neuronal damage |
Neurons![]() Created in BioRender. S, G. (2025) https://BioRender.com/ab7uib8 |
TRPV4 mediates Ca influx to neurons through protein kinase. During epileptic seizures, this results in abnormal apoptosis, while TRPV4 blockade can alleviate neuronal injury through Nf-κB. TRPV4 activation also increases nNOS levels, leading to neurotoxicity. Neuronal stiffness is enhanced by tau protein. Piezo1 signaling also plays a role in stem cell differentiation into neurons vs. astrocytes |
Oligodendrocytes![]() Created in BioRender. S, G. (2025) https://BioRender.com/ab7uib8 |
Mechanosensing modulates the myelination capacity of oligodendrocytes |
Schwann cells![]() Created in BioRender. S, G. (2025) https://BioRender.com/ab7uib8 |
The mechanical rigidity of the extracellular matrix affects Schwann cell transcription |
AD AND MECHANICAL FORCES
Research has increasingly focused on how AD affects the mechanical properties of brain tissue, such as stiffness and viscoelasticity, which may play a role in disease progression. A study utilized MRE to quantify the mechanical properties of the cerebral cortex in vivo, comparing AD patients with cognitively healthy older adults[14]. It found altered viscoelastic properties in AD patients, including increased brain stiffness, perhaps linked to neuronal loss and atrophy. These mechanical changes might contribute to the structural and functional deficits observed in AD, offering a new perspective on disease mechanisms.
Feigin et al. proposed that cumulative mechanical stress within brain tissues may co-drive brain atrophy in AD[15]. Using numerical modeling and data from existing publications, they estimated intracranial pressure equivalents of 5.92 mmHg in AD patients, compared to 3.43 mmHg in normal aging, and concluded that mechanical stress, possibly impacted by dampened arterial pulse waves, may promote pathological brain aging. Thus, changes in the brain’s mechanical environment in AD might affect both glial and neuronal health, though the exact causal relationships require further investigation.
Beyond pathophysiology, mechanical forces may also have therapeutic implications. Clinical studies have examined mechanical interventions such as whole-body vibration (WBV), transcranial ultrasound stimulation (TUSS), and auditory stimulation (AS), particularly in terms of their effects on cognitive function and AD pathology. A systematic review by Monteiro et al., which analyzed 37 papers, focused on all three modalities[16]. WBV studies reported improvements in balance and functional mobility, with some evidence of cognitive benefits, particularly at frequencies around 30 Hz, although the longer-term effects and optimal treatment protocols remain obscure. TUSS studies[17] demonstrated that MR-guided focused ultrasound can safely open the blood-brain barrier in AD patients, potentially enhancing drug delivery or amyloid-beta clearance. Rezai et al. reported reduced amyloid-beta levels in the hippocampus following noninvasive blood-brain barrier opening, hinting at a therapeutic role for ultrasound[18]. However, findings regarding cognitive outcomes are mixed, with some studies showing enhanced memory networks, while others found no significant changes in amyloid-beta levels.
Mechanical stress in biological tissue is the deformation of tissue due to physical interaction with stress factors. Increased levels of mechanical stress contribute to the development of neurodegenerative diseases, including AD[19]. The deleterious stress can originate both externally and internally. For example, the aggregation of amyloid plaques, a typical AD hallmark feature, has been observed in the brains of individuals following significant brain injuries[20]. Under similar conditions, the concentrations of soluble amyloid beta (Aβ, amyloid-β) in the cortex have been found to increase[21]. Additionally, the clearance and metabolism of amyloid were disturbed in an organism that suffered cranial injury[22]. Similar correlations have been observed with the levels of τ (tau), another typical AD factor[23]. Comparable results were also observed in laboratory experiments modeling various types of brain injuries, such as focal injury in mice[24] or fluid percussion in rats[25].
FACTORS OF MECHANICAL STRESS
External factors contributing to mechanical stress include various injuries, whether singular or occurring repeatedly. Approximately 30% of traumatic brain injury (TBI) cases exhibit a typical AD symptom - amyloid plaques[26]. These injuries cause hypoxia, a shift in the balance of pro- and anti-apoptotic processes, and inflammation, among others. Moderate TBI is prominent among professional sportsmen with frequent head traumas[27]. Moderate chronic TBIs lead to effects similar to those observed in AD[28]. Growing evidence suggests that chronic TBI can lead to AD, primarily mediated by chronic traumatic encephalopathy[29] - football players, who often suffer from encephalopathy, are five times more likely to develop AD[30]. Several publications have highlighted the link between encephalopathy and AD[31], suggesting that they may represent different stages of the same disease.
Internal factors, on the other hand, are more diverse and often less obvious. Internal stress includes the leakage of fluids from blood vessels into surrounding brain tissue and vascular injury, both of which are also features of AD[32]. The abnormal accumulation of fluids imposes a mechanical burden on surrounding brain tissues. According to Wostyn et al., mechanical stress within the brain can lead to AD-like symptoms[33]. The accumulation of tau and amyloid aggregates exerts mechanical pressure within the brain[19], and intriguingly, elevated mechanical stress increases the concentrations of amyloid-β, τ, and α synucleins[34]. Prevost et al. demonstrate that nearly any abnormal mechanical force within the brain is detrimental and damages the brain tissue[35]. Therefore, treatments aimed at alleviating mechanical stress could be beneficial.
RESPONSE TO MECHANICAL STRESS
Mechanosensing refers to the ability of cells or tissues to detect and respond to mechanical stress. Mechanotransduction is the process by which mechanical stimuli are converted into biological signals. These two phenomena are tightly related, and the review explores their relationship.
Virtually every cell type in the brain responds to the mechanical input in some form, including glial cells[36] and neurons[37]. The myelination capacity of oligodendrocytes is affected by mechanosensing[38], while astrocytes’ mechanosensitivity is linked to cardiac rhythm and metabolism[39]. Even in the peripheral nervous system, Schwann cell transcription is modulated by the mechanical rigidity of the extracellular matrix[40].
Hlavac and VandeVord found that astrocytes react to mechanical cues in multiple ways[41]. For instance, astrocytes proliferate more in a stiffer medium and increase tissue stiffness around glial scars[42]. During inflammation, Piezo1 expression in astrocytes rises[43], and their mechanosensing capability may play a key role in many therapeutic approaches[44]. Additionally, changes in myelination were observed before a delayed astrocyte response, with Piezo1 being involved in ATP release[45]. Furthermore, mechanosensing in astrocytes influences hippocampal structure and neurogenesis, ultimately affecting cognition and higher brain functions. In optic nerve experiments, astrocytes responded to mechanical input through Piezo1 activation[46], which has been implicated in glaucoma as a damaging factor[47]. The key mechanosensing cell type in the brain is microglia[48]. Key components involved in the organism’s response to mechanical input include potassium channel family members (e.g., K TREK)[49], the highly calcium-selective TRPV family of transient receptor potential cation channels[50], and the pore-forming subunit of the mechanosensitive calcium channel Piezo1[51]. Neumann et al. found that concussive traumas activate microglia, with activation persisting even after complete recovery, which may correlate with the relationship between TBI and AD[52]. Microglial activity is also induced by increased arterial stiffness - a typical sign of amyloid deposition in AD[53]. Amyloid-β increases the stiffness of the tissues it contacts. However, research has shown that the brain as a whole is less stiff by AD than a healthy brain[54]. In that research, the brain stiffness was estimated using PIB - amyloid ligand. The authors noted that among cognitively normal controls, stiffness was the same in PIB-positives and -negatives. Therefore, amyloid alone is not sufficient to alter the stiffness of the organ. The overall surge in stiffness might be the effect of cytoarchitectural wreckage, which has a closer link to tau.
Hemonnot et al. discuss recent views on microglia’s role in AD[55]. They emphasized the involvement of the NLRP3 inflammasome complex, specifically the interaction with the apoptosis-associated Speck-like (ASC) adaptor. Although KCNN4 was proposed as a therapeutic target for AD more than a decade ago[56], a recent work by Ran et al. showed that PIEZO-mediated mechanosensation triggers KCNN4-dependent potassium efflux, leading to NLRP3 inflammasome activation and subsequential pyroptosis[57]. While apoptosis has long been considered a form of non-inflammatory cell death, pyroptosis is distinctly proinflammatory, characterized by the release of proinflammatory cytokines and cellular content. In microglia, the link between these processes is facilitated by lysophosphatidylcholine, which initiates NLRP3- and NLRC4-dependent inflammasome activation[58]. Furthermore, NLRC4 is an actor in both apoptosis and pyroptosis in microglia following ischemic stroke, by promoting the release of inflammasome elements[59] [Figure 1].
Figure 1. In microglia, the mechanosensitive PIEZO1 channel responds to stiffness and shear stress by activating, leading to calcium influx. This influx causes membrane hyperpolarization and potassium efflux through KCNN4, promoting inflammasome activation. Created in BioRender. S, G. (2025) https://BioRender.com/9b9433o.
APOPTOSIS PATHWAYS
The recent developments related to Piezo1’s role in pathologies have been exhaustively covered by Lai et al.[60]. Piezo1 is widely expressed in various tissues, from blood to microglia, and is sensitive to mechanical cues from both inside and outside of the cell. It takes part in immune response and can affect gene transcription and morphological changes downstream. Ran linked the assembly of pro-apoptotic NLRP3 inflammasomes to the cell’s response to mechanical cues through pathways involving shear stress and stiffness sensing of Piezo1[61]. Furthermore, the involvement of Piezo1 pathways extends to KCNN4, a potassium-mediated calcium signaling channel essential for caspase cascade activation[57]. NLRP3 inflammasome contains components (including caspase-1) that trigger apoptosis and/or pyroptosis and has long been associated with AD[62]. Kabigting and Toyama concluded that Yes-associated protein (YAP) might mediate a cell’s response to mechanical stimuli by modulating caspase-3 expression under different mechanical conditions (e.g., medium rigidity or induced mechanical stress, stretching, compression)[63].
In AD, microglia are responsible for phagocytosing amyloid-β plaques[64]. Keren-Shaul et al. described a distinct microglia subtype - disease-associated microglia (DAM) - which played an important role in mitigating AD[65]. On the other hand, the same microglia can exert a proinflammatory effect depending on various factors, such as the nature of the stimulus, its strength, and persistence[66]. Yin et al. claim that such microglia can be the principal source of neuroinflammation in AD[67].
Transient receptor potential cation channel subfamily V member 2 (TRPV2) belongs to the TRP channel family, which facilitates transmembrane communication through ion transport and responds to stimuli such as high temperatures, osmotic changes, and mechanical forces[68]. Inhibition of TRPV2 has been shown to reduce cognitive impairments associated with amyloid pathology[69]. Enrich-Bengoa et al. reported that TRPV2 is broadly expressed in microglia cell bodies, and its expression was reduced with anti-inflammatory treatment[70].
Microglia respond to changes in mechanical stiffness via Piezo1 channels[71], to relieve the burden of amyloid plaques[72] - a property that fatty acids can hinder[73]. Although Piezo1 mediates microglial responses to mechanical stimuli, intriguingly, the lower the level of Piezo1 in microglia, the more motile microglia are toward amyloid plaques. Zhu et al. found that Piezo1-deficient amoeboid microglia are the ones that ultimately encircle amyloid plaques[74], despite earlier findings by Bouvier et al., which showed that microglia upregulate Piezo1 in response to amyloid plaques[75]. This apparent paradox may be explained by the involvement of alternative pathways regulating microglial migration, such as KCNN4[76]. Furthermore, Liu et al. advocate that frequent or excessive activation of Piezo1 may itself induce inflammation[77], contributing to a vicious cycle of pathological progression[78].
OXIDATIVE STRESS IN MECHANOSENSING
The permanent and accumulating presence of foreign bodies, such as amyloid-β plaques, within the brain tissue exerts prolonged mechanical stress, evokes a deleterious inflammatory response, leads to oxidative stress, and thus provokes further aggregation of various proteins[79], including amyloid-β. Increased oxidative stress has been identified as an important feature in both TBI and AD[80], which further suggests a hidden link between the two. Oxidative stress can, directly and indirectly, modulate the activity of mechanosensitive channels, influencing their gating properties and downstream signaling. It damages cellular components, including lipids, proteins, and DNA, and is implicated in neuronal death and dysfunction. Oxidative stress is also one of the key factors for artery stiffening[81], which is one of the principal sources of mechanical stress observed in AD pathology. Increased oxidative stress is observed in AD and leads to the formation of the NLRP3 inflammasome[82], which subsequently triggers apoptosis and also interacts with mechanosensing pathways. TRPV4 activation by Aβ in hippocampal cells can increase calcium influx, leading to more oxidative stress and cell death, worsening AD[83]. Activation of TRPV4 enhances oxidative stress by inhibiting antioxidant enzymes such as catalase (CAT) and glutathione peroxidase (GSH-Px) and increasing neuronal nitric oxide synthase (nNOS), leading to neurotoxicity[84]. TRPV4’s role in astrocytic calcium signaling suggests it may disrupt glial support functions under oxidative stress, while Piezo1’s role in microglial mechanosensitivity could alter their response to Aβ, potentially leading to a proinflammatory state exacerbated by reactive oxygen species (ROS). This suggests that in AD, TRPV4 activity may be amplified. A study on endothelial cells under high glucose conditions also found that Piezo1 activation with the agonist Yoda1 significantly increased intracellular ROS levels[85]. Finally, mechanosensitive Piezo1 expression is upregulated with age and in AD, provoked by amyloid-β plaques[86] and mediates the reduction of the oxidative stress effect and biological aging in intervertebral discs. Wang et al. observed that an increase in the elasticity modulus of the extracellular matrix led to enhanced Piezo1 expression, accompanied by apoptosis and cellular senescence[87]. This caused calcium influx and, consequently, the surge of reactive oxygen species. They also observed that the expression of GRP78 and CHOP is also upregulated. They reinforced these results with experiments on rats, where silencing or completely deactivating Piezo1 reduced the elasticity modulus and rescued tissues.
MECHANICAL STRESS AND NERVOUS TISSUE
The consequence of mechanical force includes the activation of several related pathways such as p38/MAPK[88], caspases[89], the proinflammatory response of microglia[90], and higher levels of oxidative stress, eventually increasing the likelihood of apoptosis[91]. Hsieh et al. triggered cancer cell self-destruction via epigenetic p38/MAPK modifications[92]. The action of these pathways is not limited to apoptosis; PI3K (microglia tool for neuroinflammation moderation[93]) and apoptosis-related Erk 1/2 MAPK pathways are also launched through mechanosensing. Tissue and force types determine the balance of these pathways, resulting in a spectrum of results[94]. Piezo1 is a key player in an organism’s response to mechanical input, functioning not in a simple binary manner, but as an indicator of the organism’s efforts to restore homeostasis. For example, in the brain and other neuronal tissues, astrocytes and microglia carry out phagocytosis to clean garbage[95], including amyloid-β and dead neurons. Piezo1 removal in astrocytes reduced the hippocampal volume and overall brain mass, cutting the production of neurons, which affected cognition and mental function in the long term, while increased expression of Piezo1 significantly enhanced these functions[96]. In neurons, there is a group of publications that connect Piezo1 to apoptosis. In an experiment with oxygen-glucose mediated apoptosis, activation of Piezo1 increases calcium influx, while its inhibition decreases it[97]. Calpain activation, which is part of the apoptosis pathway, follows intracellular calcium fluctuations, suggesting a link between the Piezo1 mechanosensitivity pathway and apoptosis. Gain-of-function mutations in TRPV4 can decrease calpain activity while increasing adhesion[98]. The experiment showed TRPV4 was expressed in microglia in response to mechanical stimulation with ultrasound, and this boosted apoptosis rates, while TRPV4 blockage protected against it[99]. Thus, Piezo1 is not the only determinant of the final effect of calcium role; the eventual health or pathology of the entire organism is a multifaceted problem.
Microglial apoptosis induced by ROS bears similarity to cell death due to mechanosensitivity in other cell types such as endothelial and muscle cells[100] and lung tissue[101], and is related to the oxidative stress response in microglia[102].
KEY MOLECULES IN THE MECHANOSENSITIVE PATHWAY
Amyloid-β plaques are stiffer than the brain tissue[103]. What is worse, the smaller the amyloid-β aggregates, the stiffer they are[104]. This could mean that in the early stages of AD, the higher number of dispersed plaques is more “unsettling” for the brain. The rigidity of tau, on the other hand, is modulated by the strain rate[105] and phosphorylation[106]. Due to their different cellular localization, tau can increase the stiffness of neuronal cells[107], while amyloid-β increases the membrane stiffness[108]. So, amyloid-β increases the stiffness, but the stiffness in turn exacerbates the amyloid-β burden. In AD, the brain stiffens unevenly, with higher rates of stiffening in the frontal, parietal, and temporal lobes[109]. Tau can also stiffen the blood vessels, impairing blood flow[110].
Transient receptor potential vanilloid type 4 (TRPV4) is a mechanosensitivity-related ion channel. TRPV4 not only regulates microglia activity[111], but also functions as a differential sensor in the retina to mechanical input[112]. Blocking TRPV4 reduces oxidative stress[113]. TRPV4 activation can be changed with phosphorylation[114]. TRPV4 activation/silencing with siRNA/agonist demonstrated the drop/increase in cholesterol levels, while dampening the ABCA1 transporter, responsible for cholesterol flow, resulting in cholesterol not exiting the cell and a lift of tau protein phosphorylation[115]. TRPV4 activation leads to calcium flow into the cell. Calcium binds to calmodulin, activating NO synthase, which generates nitric oxide and leads to vasodilation. Amyloid-β stiffens arteries and interferes with vasodilation, which might be mediated with TRPV4[116]. Vasodilation can lead to reduced blood pressure in sectors of the blood system, which induces ischemia[117], resulting in blood vessel degeneration[118] and subsequent liquid accumulation in the brain with associated mechanical pressure, typical of AD[119]. This means TRPV4-related dysfunction can be at least partially responsible for these unfavorable consequences. Stiffness activates TRPV2 channels and impacts apoptosis[120], intimately related to the aforementioned TRPV4[121]. TRPV4, Piezo1, and KCNN4 are all ion channels sensitive to mechanical cues. Richardson raises the question of why the body needs several pathways, and demonstrates that they might serve complementary functions: Piezo1 responds to both cell compression and membrane stretch, while TRPV4 is activated only by deflections[122].
ION CHANNELS ROLES
Piezo1 is triggered by alterations in membrane tension[123]. Its sensitivity can be modulated by the ambient substrate of the cell[124]. Additionally, Piezo1 transduces mechanosensitivity signals that can determine the fate of stem cells to become either neurons or astrocytes[125]. Moreover, Piezo1 can respond to the cell’s internal mechanical stimuli, migrating along the plasma membrane to accumulate near force sources and forming localized calcium microdomains[126].
KCNN4 is a calcium-activated potassium channel that is coupled, among others[127], to TRPV4 and, potentially, Piezo1. TRPV2 and TRPV4 regulate microglia function[128], with a potential to reduce the microglia inflammation. While microglia can help clear amyloid-β, it can also damage neurons when activated. Under hypothermic conditions, this unfavorable property of microglia was hindered, along with proinflammatory cytokines. Treated with both TRPV4 agonist and antagonist, microglia demonstrated corresponding reduction and increase in neuronal damage caused. Thus, Fukuda et al. identified a crucial role for the TRPV4-AMPK-NF-κB pathway (where NF-κB, which normally induces apoptosis, is phosphorylated via the AMPK hypothermia-regulating pathway)[129]. Previously, hypothermic conditions, i.e., low-temperature treatment, were shown to protect from loss of synapses in neurodegenerative diseases (AD included) and, thus, against pathological symptoms, through boosted activation of RMB3[130].
MSA
MSA is a phenomenon observed across multiple tissues and is particularly significant given the conserved nature of apoptosis across different cell types[131]. Calpains, which are involved in numerous cellular processes, play a role in MSA. In vascular muscle cells, MSA is associated with elevated p53 protein expression and can be moderated by calpain activity[132]. However, age-related changes, particularly those involving oxidative stress, disrupt normal regulation, causing excessive calpain activation, which renders calpains pro-apoptotic contributors in AD[133]. In epithelium tissues, mechanical forces activate the RhoA signaling pathway, which triggers apical cell extrusion (a process functionally analogous to “apoptosis” in epithelial cells)[134]. This extrusion is regulated by Piezo1 channels[135]. Inhibition of Piezo1, either through genetic knockdown or pharmacological blockade using gadolinium, impairs cell extrusion and leads to excessive accumulation of epithelium cells.
In endothelium cells, mechanotransduction activates apoptotic and inflammatory signaling pathways, resulting in atherosclerosis. This process occurs in the arteries where blood flow becomes non-laminar, such as at bends or bifurcations, leading to the activation of pro-apoptotic signaling through various pathways. In contrast, laminar flow promotes the expression of anti-inflammatory genes[136]. Kawaue et al. conducted experiments on kidney cells and found that mechanotransduction is not a mere “on/off switch” for apoptosis[137]. Instead, the apoptosis rate varies proportionately with changes in mechanical stress. This process is mediated by YAP, which interacts with CDK6 signaling and has been implicated in preventing cognitive decline in AD[138]. Reduced YAP levels - sequestered by cytoplasmic amyloid-β plaques - have been associated with increased necrosis in AD and may play a role in necrosis processes[139]. YAP serves as a convergence point for the TRPV4 and Hippo pathways through membrane mechanosensing mechanisms[140]. TRPV4 mediates calcium influx, which, when excessive, elevates apoptosis rates beyond healthy levels. This phenomenon has been observed in cortical neurons during epileptic seizures[141], where calcium influx is regulated by protein kinase C (PKC) signaling cascades. Epileptic seizures are common in AD patients[142] and have been linked to mechanosensitive ion channels and oxidative stress[143]. Many types of epileptic seizures are accompanied by elevated blood pressure[144], which might act as a mechanical stimulus activating the brain’s mechanosensing pathways in AD. The PKC cascade also activates calpain and caspases; in the hippocampus, astrocyte and microglia death during induced epileptic seizures is mediated by the NLRP3 inflammasome and/or TNF-α release[145]. In a murine epilepsy model, TRPV4 inhibition alleviated hippocampal neuronal injury and inflammation by modulating Nf-κB signaling[146].
In AD, pathologic tau mainly aggregates intracellularly (as opposed to amyloid-β, which accumulates in the intercellular space). Caspase-3 has been shown to cleave tau[147], promoting its co-localization in the corpus callosum and contributing to neurodegeneration[148]. Donnaloja et al. compiled evidence linking tau to mechanotransduction pathways[149]. When tau aggregates into tangles within cells, it might interfere with transduction pathways, leading to cognitive impairment. Their findings suggest that tau undergoes hyperphosphorylation in response to nuclear blebbing and invaginations, events driven by lamin A - a component involved in extracellular matrix-related mechanotransduction. Niethammer reviewed how calcium signaling is disrupted by nuclear stiffening: activation of the mechanosensitive channel Piezo1, in response to mechanical stretch, is influenced by lamin A-mediated nuclear stiffness, which in turn enhances intracellular calcium release[150]. Lomakin et al. demonstrated that the nuclear envelope and its stress-sensing proteins regulate cellular deformability[151]. When mechanical stress exceeds a critical threshold, the nuclear envelope initiates calcium release. Calcium ions then modulate nuclear deformation, softening, and the cell’s adaptive response to mechanical stress[152]. Given that tau stiffening due to phosphorylation is well established[106], it is plausible that phosphorylated tau contributes to Piezo1-mediated nuclear signaling, potentially acting as a mechanosensitive trigger of apoptosis in AD. Moreover, even extracellular tau appears to affect nuclear integrity. Sun et al. showed that exposure to extracellular tau can induce nuclear invaginations[153].
CERAMIDES AND APOPTOSIS
Ceramides are pro-apoptotic molecules[154]. When stimulated by oxidative stress in AD, ceramides promote apoptosis and contribute to the production of amyloid-β[155]. Ceramides also modulate the MAPK signaling pathway[156] and reduce Bcl-2 levels, which is typically an anti-apoptotic protein under normal conditions[157]. Interestingly, Bcl-2 itself regulates ceramide levels[158]. Integrins and adhesion proteins respond to mechanical forces within the organism[159], with TRPV4 playing a key role in integrin activation[160]. In the hippocampus, TRPV4 activates apoptosis through the silencing of the PI3K/Akt pathway (related to oxidative stress[161]) and by promoting p38/MAPK activation[162].
Sphingomyelinases, which are abundant in nervous tissue, play a crucial role in ceramide production and are implicated in many neurological disorders[163]. These enzymes can deactivate Piezo1 channel suppression[164], thereby enabling prolonged activation and increasing sensitivity to mechanical force. Although primarily studied in the endothelium, this mechanism may also apply to other tissues. Piezo1 activity is inhibited by SMPD3 sphingomyelinase in response to pressure. The lipids resulting from sphingomyelinase activity form an environment conducive to the fast inactivation of Piezo1, enhancing its ability to sense mechanical input in the endothelium[165].
Current research on mechanosensing and ceramides is primarily limited to endothelial cells, where cyclic mechanical stress has been shown to directly trigger ceramide production[166]. The mechanosensing-mediated production of ceramide involves membrane caveolae[167], which are formed in the presence of caveolin[168]. Initially, the function of caveolae was thought to be restricted to endothelium and adipose tissues. However, recent research has indicated that they also play a role in nervous tissue[169], and can even alter the morphology of microglia[170]. Caveolin-1 exhibits both pro- and anti-apoptotic traits[171], and its expression is increased in AD[172]. Caveolins seem to be associated with mental disorders induced by oxidative stress[173] and studies have demonstrated that they respond to ROS levels[174]. Thus, caveolins may serve as a connection between mechanosensing, oxidative stress, and ceramides [Figure 2].
Figure 2. Ceramides interact with the MAPK pathway, which is associated with apoptosis. They also partake in amyloid formation. Ceramides are generated in response to mechanical stress through the mediation of caveolae and sphingomyelinase. The formation of caveolae under mechanical stress may be mediated by oxidative stress. Created in BioRender. S, G. (2025) https://BioRender.com/ab7uib8.
HIPPO PATHWAY AND MECHANOSENSING
Researchers have explored the role of the Hippo pathway - primarily responsible for regulating cell proliferation and controlling organ growth - in AD and aberrant apoptosis[175]. The Hippo pathway acts in microglia cells, helping to mitigate inflammation caused by amyloid-β[176]. A human homolog of Hippo, macrophage-stimulating protein MST1, has been shown to decrease neuronal apoptosis[177] when suppressed, potentially through the involvement of oxidative stress[178]. Some studies[179] suggest that the activity of the Hippo pathway does not correlate with caspase levels, indicating the presence of multiple mechanisms driving apoptosis.
In the blood system, discoidin domain receptor 1 (DDR1) mediates YAP activation in response to both mechanical and chemical stimuli. DDR1 also regulates LATS1 phosphorylation in a liquid-liquid phase separation-dependent manner[180]. As a result, DDR1 antagonizes the Hippo pathway and contributes to arterial stiffening. The LATS1/YAP interaction has been shown to reduce neuronal apoptosis in rats[181]. BIN1 is an intrinsically disordered protein (IDP)[182]. Moreover, BIN1 binds to tau protein through its SH3 domain, which likely prevents tau aggregation. The endocytosis of extracellular tau and the membrane-bound proteins BACE and amyloid-β are significant contributors to AD, and BIN1 is involved in these processes[11]. Specifically, AD is characterized by a decreased expression of the neuronal isoform of BIN1 compared to its ubiquitous isoform[183].
BIN1, which is strongly implicated in AD[184], is a member of the BAR domain family, known for its ability to sense membrane curvature[185]. Consequently, BIN1 also plays a role in mediating mechanical responses at the cellular level. Through its BAR domains, BIN1 influences the function of actin filaments[186]. Associated proteins can increase the concentration of G-actin, which, in turn, triggers polymerization[187]. This process leads to the conversion of G-actin into F-actin[188]. F-actin has been shown to exhibit inverse co-localization with stress granules (SG), effectively avoiding them[189]. Therefore, it is hypothesized that SG are more abundant in regions with low F-actin levels. This hypothesis is partially proven with experiments on fibroblasts, where apoptosis was induced by mechanical force via Piezo1 activation and destruction of the actin cytoskeleton[190]. In dendritic spines, amyloid-β was observed to mediate the de-polymerization of F-actin back to G-actin[191]. SG contain DDX3X, which triggers the activation of the NLRP3 inflammasome[192]. The role of the Hippo pathway is summarized in Figure 3.
Figure 3. The Hippo pathway in humans begins with MST1. Downstream of MST1 is LATS1, which interacts with YAP to reduce apoptosis. LATS1 is also linked to SAV1, which interacts with FlnA in response to mechanical force. FlnA might alter Piezo1 sensitivity and, consequently, mechanical input, thereby reducing apoptosis. This appears to form a feedback loop with SAV1, which is sensitive to mechanical force, as previously mentioned. Created in BioRender. S, G. (2025) https://BioRender.com/ab7uib8. YAP: Yes-associated protein; SAV1: salvador homolog 1; FlnA: filamin A.
CERAMIDES IN MECHANOSENSING
G3BP1 and G3BP2 are widely implicated in neurodegeneration[193]. These paralogous proteins participate in the formation of SG[194]. In cancer, G3BP1 is associated with mRNA partitioning, which refers to the breakdown of specific transcripts into polysomes - ribosomes with mRNA molecules attached. It responds to oxidative stress by transcribing mRNAs related to protein complexes that later form the SG[195]. Feng et al. showed that G3BP2 has a lower mechanobinding capacity with filamin A (FlnA) compared to G3BP1[196]. Gottlieb hypothesized that FlnA might reduce stress, thereby dampening Piezo1 channel sensitivity to mechanical stress, as evidenced by significantly increased Piezo1 activity in FlnA knockout models[197]. Salvador homolog 1 (SAV1), a component of the Hippo pathway, interacts with FlnA in response to mechanical force. This interaction increases apoptosis in the murine liver[198].
Wei et al. reported that G3BP2 is part of a signaling pathway connected to TWIST1[199]. In mice with TWIST1-induced craniosynostosis, intracranial pressure was significantly elevated[200], providing evidence of its engagement in organic mechanosensitivity. Yang et al. found a similar effect with G3BP2 and G3BP, whereby SG assemble in G3BP1-knockout cells with RNA-recognition motifs of hnRNPA1 and hnRNPA2B1, suggesting a compensatory mechanism[201]. This finding is crucial for further investigation, as TWIST1 can regulate gene silencing, at least in lung cancer cells[202] and may serve as another regulatory switch linking TWIST1 to mechanosensing capacity, similar to MAG.
CONCLUSION
This review summarizes current perspectives on the role of mechanosensing in the progression of amyloid-β-associated pathologies, with a particular focus on AD. Growing attention has been directed toward understanding how tissue mechanosensing contributes both to the damage caused by AD and to protective mechanisms. Theoretical studies have provided compelling evidence that mechanosensing - primarily mediated by the Piezo1 channel - may be a key factor in AD pathology. Moreover, this mechanosensitive property could function as a regulatory switch, enabling the organism to recuperate to varying degrees. However, the full mechanistic pathway remains largely unexplored experimentally. One possible reason for this is the challenge of applying long-term mechanical stimulation to nervous tissue while monitoring outcomes. Among the pathways involved, the Hippo pathway stands out for its dual role in apoptosis and mechanotransduction. In this review, we have also highlighted several other genes that appear underrepresented in the literature on mechanosensing. We further attempted to construct a coherent theoretical framework connecting mechanosensing, oxidative stress, and apoptosis in the context of AD.
To deepen the understanding, it is crucial to dissect the cell-specific mechanisms of mechanosensing, with a focus on AD, and the interactions between different cell types. Longitudinal studies employing techniques such as magnetic resonance elastography are essential for tracking dynamic changes in brain tissue stiffness and viscoelasticity over time, and for correlating these changes with cognitive decline and pathological markers. Additionally, the role of the extracellular matrix in AD-related mechanosensing dysfunction warrants thorough investigation.
At the molecular level, the precise mechanisms by which TRPV4 and Piezo1 interact in AD - and how these interactions influence calcium signaling, oxidative stress, and apoptosis - represent a promising avenue for further research. The role of post-translational modifications, such as phosphorylation, in regulating these channels is another area of inquiry, as are the triggers and signaling pathways leading to NLRP3 inflammasome activation in microglia. The relationships among ROS, ceramides, and caveolae, along with the role of BIN1 in stress granule formation - currently presented in this manuscript as tentative - would benefit from experimental verification. The therapeutic potential of targeting these molecular “nodes”, as well as the Hippo pathway, particularly for modulating microglial responses and neuronal apoptosis, gives promises, albeit still at a strategic and exploratory stage horizon. For example, vitagenes (e.g., Nrf2, regulatory proteins, and enzymes capable of producing H2S) have been shown to protect astrocytes from oxidative stress[203]. Their potential relevance in regulating key mechanisms involved in inflammation and neurodegenerative damage may be linked to mechanosensitive properties, and possibly to chemoprevention. As such, their connection to ceramides and the ion channels discussed in this manuscript may reveal important insights.
From a preventive standpoint, the development of biomarkers for mechanical stress and mechanosensing dysfunction could support early diagnosis and monitoring of disease progression.
DECLARATIONS
Authors’ contributions
Made substantial contributions to the conception and design of the study: Sapozhnikov G
Provided essential review and remarks: Ranganathan R
Provided administrative, technical, and material support and supervision and guidance: Wang S, Song YQ
Availability of data and materials
Not applicable.
Financial support and sponsorship
This work was supported by a post-graduate scholarship from the University of Hong Kong.
Conflicts of interest
All authors declared that there are no conflicts of interest.
Ethical approval and consent to participate
Not applicable.
Consent for publication
Not applicable.
Copyright
© The Author(s) 2025.
REFERENCES
1. Alzheimer’s Association. 2024 Alzheimer’s disease facts and figures. Available from: https://wehco.media.clients.ellingtoncms.com/news/documents/2024/03/19/Alzheimers_report.pdf. [Last accessed on 27 Apr 2025].
2. Wong W. Economic burden of Alzheimer disease and managed care considerations. Am J Manag Care. 2020;26:S177-83.
3. Deschaintre Y, Richard F, Leys D, Pasquier F. Treatment of vascular risk factors is associated with slower decline in Alzheimer disease. Neurology. 2009;73:674-80.
4. Rasmussen J, Langerman H. Alzheimer’s disease - Why we need early diagnosis. Degener Neurol Neuromuscul Dis. 2019;9:123-30.
5. Ittner LM, Götz J. Amyloid-β and tau - a toxic pas de deux in Alzheimer’s disease. Nat Rev Neurosci. 2011;12:65-72.
6. Spires-Jones TL, Hyman BT. The intersection of amyloid beta and tau at synapses in Alzheimer’s disease. Neuron. 2014;82:756-71.
7. Pimenova AA, Raj T, Goate AM. Untangling genetic risk for Alzheimer’s disease. Biol Psychiatry. 2018;83:300-10.
8. Sochocka M, Zwolinska K, Leszek J. The infectious etiology of Alzheimer’s disease. Curr Neuropharmacol. 2017;15:996-1009.
9. Jiang C, Li G, Huang P, Liu Z, Zhao B. The gut microbiota and Alzheimer’s disease. J Alzheimers Dis. 2017;58:1-15.
10. Shandilya S, Kumar S, Kumar Jha N, Kumar Kesari K, Ruokolainen J. Interplay of gut microbiota and oxidative stress: perspective on neurodegeneration and neuroprotection. J Adv Res. 2022;38:223-44.
11. Ranganathan R, Sapozhnikov G, Ni W, Li S, Song Y. Recent developments in the role of DNA damage response and understanding its implications for new therapeutic approaches in Alzheimer’s disease. Transl Med Aging. 2023;7:52-65.
12. Kinney JW, Bemiller SM, Murtishaw AS, Leisgang AM, Salazar AM, Lamb BT. Inflammation as a central mechanism in Alzheimer’s disease. Alzheimers Dement. 2018;4:575-90.
13. Rajendran L, Paolicelli RC. Microglia-mediated synapse loss in Alzheimer’s disease. J Neurosci. 2018;38:2911-9.
14. Hiscox LV, Johnson CL, McGarry MDJ, et al. Mechanical property alterations across the cerebral cortex due to Alzheimer’s disease. Brain Commun. 2020;2:fcz049.
15. Feigin VL, Roth GA, Naghavi M, et al; Global Burden of Diseases, Injuries and Risk Factors Study 2013 and Stroke Experts Writing Group. Global burden of stroke and risk factors in 188 countries, during 1990-2013: a systematic analysis for the Global Burden of Disease Study 2013. Lancet Neurol. 2016;15:913-24.
16. Monteiro F, Sotiropoulos I, Carvalho Ó, Sousa N, Silva FS. Multi-mechanical waves against Alzheimer’s disease pathology: a systematic review. Transl Neurodegener. 2021;10:36.
17. Lipsman N, Meng Y, Bethune AJ, et al. Blood-brain barrier opening in Alzheimer’s disease using MR-guided focused ultrasound. Nat Commun. 2018;9:2336.
18. Rezai AR, Ranjan M, D’Haese PF, et al. Noninvasive hippocampal blood-brain barrier opening in Alzheimer’s disease with focused ultrasound. Proc Natl Acad Sci U S A. 2020;117:9180-2.
19. Levy Nogueira M, Epelbaum S, Steyaert JM, Dubois B, Schwartz L. Mechanical stress models of Alzheimer’s disease pathology. Alzheimers Dement. 2016;12:324-33.
20. Roberts GW, Gentleman SM, Lynch A, Murray L, Landon M, Graham DI. Beta amyloid protein deposition in the brain after severe head injury: implications for the pathogenesis of Alzheimer’s disease. J Neurol Neurosurg Psychiatry. 1994;57:419-25.
21. DeKosky ST, Abrahamson EE, Ciallella JR, et al. Association of increased cortical soluble abeta42 levels with diffuse plaques after severe brain injury in humans. Arch Neurol. 2007;64:541-4.
22. Graham DI, Gentleman SM, Nicoll JAR, et al. Altered β-APP metabolism after head injury and its relationship to the aetiology of Alzheimer’s disease. In: Baethmann A, Kempski OS, Plesnila N, Staub F, editors. Mechanisms of secondary brain damage in cerebral ischemia and trauma. Vienna: Springer; 1996. pp. 96-102.
23. Zanier ER, Zoerle T, Fiorini M, et al. Heart-fatty acid-binding and tau proteins relate to brain injury severity and long-term outcome in subarachnoid haemorrhage patients. Br J Anaesth. 2013;111:424-32.
24. Cribbs DH, Chen LS, Cotman CW, LaFerla FM. Injury induces presenilin-1 gene expression in mouse brain. Neuroreport. 1996;7:1773-6.
25. Iwata A, Chen XH, McIntosh TK, Browne KD, Smith DH. Long-term accumulation of amyloid-beta in axons following brain trauma without persistent upregulation of amyloid precursor protein genes. J Neuropathol Exp Neurol. 2002;61:1056-68.
26. Sivanandam TM, Thakur MK. Traumatic brain injury: a risk factor for Alzheimer’s disease. Neurosci Biobehav Rev. 2012;36:1376-81.
27. Russell ER, Mackay DF, Lyall D, et al. Neurodegenerative disease risk among former international rugby union players. J Neurol Neurosurg Psychiatry. 2022;93:1262-8.
28. Arena JD, Smith DH, Lee EB, et al. Tau immunophenotypes in chronic traumatic encephalopathy recapitulate those of ageing and Alzheimer’s disease. Brain. 2020;143:1572-87.
29. Pearce AJ, Sy J, Lee M, et al. Chronic traumatic encephalopathy in a former Australian rules football player diagnosed with Alzheimer’s disease. Acta Neuropathol Commun. 2020;8:23.
30. Mackay DF, Russell ER, Stewart K, MacLean JA, Pell JP, Stewart W. Neurodegenerative disease mortality among former professional soccer players. N Engl J Med. 2019;381:1801-8.
31. Faden AI, Loane DJ. Chronic neurodegeneration after traumatic brain injury: Alzheimer disease, chronic traumatic encephalopathy, or persistent neuroinflammation? Neurotherapeutics 2015;12:143-50.
32. Stone J, Johnstone DM, Mitrofanis J, O’Rourke M. The mechanical cause of age-related dementia (Alzheimer’s disease): the brain is destroyed by the pulse. J Alzheimers Dis. 2015;44:355-73.
33. Wostyn P, Audenaert K, De Deyn PP. Alzheimer’s disease-related changes in diseases characterized by elevation of intracranial or intraocular pressure. Clin Neurol Neurosurg. 2008;110:101-9.
34. Levy Nogueira M, Hamraz M, Abolhassani M, et al. Mechanical stress increases brain amyloid β, tau, and α-synuclein concentrations in wild-type mice. Alzheimers Dement. 2018;14:444-53.
35. Prevost TP, Jin G, de Moya MA, Alam HB, Suresh S, Socrate S. Dynamic mechanical response of brain tissue in indentation in vivo, in situ and in vitro. Acta Biomater. 2011;7:4090-101.
36. Cullimore B, Baumann J, Rudzitis CN, Jo AO, Kirdajova D, Križaj D. Mechanotransduction mechanisms in central nervous system glia. Neural Regen Res. 2023;18:1031-2.
37. Stukel JM, Willits RK. Mechanotransduction of neural cells through cell-substrate interactions. Tissue Eng Part B Rev. 2016;22:173-82.
38. Makhija EP, Espinosa-Hoyos D, Jagielska A, Van Vliet KJ. Mechanical regulation of oligodendrocyte biology. Neurosci Lett. 2020;717:134673.
39. Turovsky EA, Braga A, Yu Y, et al. Mechanosensory signaling in astrocytes. J Neurosci. 2020;40:9364-71.
40. Rosso G, Wehner D, Schweitzer C, et al. Matrix stiffness mechanosensing modulates the expression and distribution of transcription factors in Schwann cells. Bioeng Transl Med. 2022;7:e10257.
41. Hlavac N, VandeVord PJ. Astrocyte mechano-activation by high-rate overpressure involves alterations in structural and junctional proteins. Front Neurol. 2019;10:99.
42. Marinval N, Chew SY. Mechanotransduction assays for neural regeneration strategies: a focus on glial cells. APL Bioeng. 2021;5:021505.
43. Yu D, Jayasi J, Womac A, Kearns A, Bae C. Astrocyte inflammatory regulation of Piezo1 mechanosensitive ion channel. Biophys J. 2022;121:493A.
44. Rocha DN, Carvalho ED, Relvas JB, Oliveira MJ, Pêgo AP. Mechanotransduction: exploring new therapeutic avenues in central nervous system pathology. Front Neurosci. 2022;16:861613.
45. Chen KH, Qiu Z. Sensational astrocytes: mechanotransduction in adult brain function. Neuron. 2022;110:2891-3.
46. Liu J, Yang Y, Liu Y. Piezo1 plays a role in optic nerve head astrocyte reactivity. Exp Eye Res. 2021;204:108445.
47. Liu Y, Liu J, Clark AF, Yang Y. Piezo1 plays a role in optic nerve head astrocyte mechanotransduction. Invest Ophthalmol Vis Sci. 2019;60:6185. Available from: https://iovs.arvojournals.org/article.aspx?articleid=2745241. [Last accessed on 27 Apr 2025].
48. Melo P, Socodato R, Silveira MS, Neves MAD, Relvas JB, Mendes Pinto I. Mechanical actuators in microglia dynamics and function. Eur J Cell Biol. 2022;101:151247.
49. Tortorella I, Argentati C, Emiliani C, Morena F, Martino S. Biochemical pathways of cellular mechanosensing/mechanotransduction and their role in neurodegenerative diseases pathogenesis. Cells. 2022;11:3093.
50. O’Neil RG, Heller S. The mechanosensitive nature of TRPV channels. Pflugers Arch. 2005;451:193-203.
51. Hill RZ, Loud MC, Dubin AE, Peet B, Patapoutian A. PIEZO1 transduces mechanical itch in mice. Nature. 2022;607:104-10.
52. Neumann KD, Seshadri V, Thompson XD, et al. Microglial activation persists beyond clinical recovery following sport concussion in collegiate athletes. Front Neurol. 2023;14:1127708.
53. Hughes TM, Kuller LH, Barinas-Mitchell EJ, et al. Arterial stiffness and β-amyloid progression in nondemented elderly adults. JAMA Neurol. 2014;71:562-8.
54. Murphy MC, Huston J 3rd, Jack CR Jr, et al. Decreased brain stiffness in Alzheimer’s disease determined by magnetic resonance elastography. J Magn Reson Imaging. 2011;34:494-8.
55. Hemonnot AL, Hua J, Ulmann L, Hirbec H. Microglia in Alzheimer disease: well-known targets and new opportunities. Front Aging Neurosci. 2019;11:233.
56. Maezawa I, Jenkins DP, Jin BE, Wulff H. Microglial KCa3.1 channels as a potential therapeutic target for Alzheimer’s disease. Int J Alzheimers Dis. 2012;2012:868972.
57. Ran L, Ye T, Erbs E, et al. KCNN4 links PIEZO-dependent mechanotransduction to NLRP3 inflammasome activation. Sci. Immunol. 2023;8:eadf4699.
58. Freeman L, Guo H, David CN, Brickey WJ, Jha S, Ting JP. NLR members NLRC4 and NLRP3 mediate sterile inflammasome activation in microglia and astrocytes. J Exp Med. 2017;214:1351-70.
59. Poh L, Kang SW, Baik SH, et al. Evidence that NLRC4 inflammasome mediates apoptotic and pyroptotic microglial death following ischemic stroke. Brain Behav Immun. 2019;75:34-47.
60. Lai A, Cox CD, Chandra Sekar N, et al. Mechanosensing by Piezo1 and its implications for physiology and various pathologies. Biol Rev Camb Philos Soc. 2022;97:604-14.
61. Ran L. Investigation of the roles of mechanical cues in NLRP3 inflammasome activation. 2022. Available from: https://theses.fr/2022STRAJ042. [Last accessed on 27 Apr 2025].
62. Feng YS, Tan ZX, Wu LY, Dong F, Zhang F. The involvement of NLRP3 inflammasome in the treatment of Alzheimer’s disease. Ageing Res Rev. 2020;64:101192.
63. Kabigting JET, Toyama Y. Interplay between caspase, Yes-associated protein, and mechanics: a possible switch between life and death?. Curr Opin Cell Biol. 2020;67:141-6.
64. Condello C, Yuan P, Schain A, Grutzendler J. Microglia constitute a barrier that prevents neurotoxic protofibrillar Aβ42 hotspots around plaques. Nat Commun. 2015;6:6176.
65. Keren-Shaul H, Spinrad A, Weiner A, et al. A unique microglia type associated with restricting development of Alzheimer’s disease. Cell. 2017;169:1276-90.e17.
66. Subhramanyam CS, Wang C, Hu Q, Dheen ST. Microglia-mediated neuroinflammation in neurodegenerative diseases. Semin Cell Dev Biol. 2019;94:112-20.
67. Yin Z, Raj D, Saiepour N, et al. Immune hyperreactivity of Aβ plaque-associated microglia in Alzheimer’s disease. Neurobiol Aging. 2017;55:115-22.
68. Kojima I, Nagasawa M. TRPV2. In: Nilius B, Flockerzi V, editors. Mammalian Transient receptor potential (TRP) cation channels. Berlin: Springer Berlin Heidelberg; 2014. pp. 247-72.
69. Thapak P, Bishnoi M, Sharma SS. Tranilast, a transient receptor potential vanilloid 2 channel (TRPV2) inhibitor attenuates amyloid β-induced cognitive impairment: possible mechanisms. Neuromolecular Med. 2022;24:183-94.
70. Enrich-Bengoa J, Manich G, Valente T, et al. TRPV2: a key player in myelination disorders of the central nervous system. Int J Mol Sci. 2022;23:3617.
71. Hu J, Chen Q, Zhu H, et al. Microglial Piezo1 senses Aβ fibril stiffness to restrict Alzheimer’s disease. Neuron. 2023;111:15-29.e8.
72. Jäntti H, Sitnikova V, Ishchenko Y, et al. Microglial amyloid beta clearance is driven by PIEZO1 channels. J Neuroinflammation. 2022;19:147.
73. Ivkovic S, Major T, Mitic M, Loncarevic-Vasiljkovic N, Jovic M, Adzic M. Fatty acids as biomodulators of Piezo1 mediated glial mechanosensitivity in Alzheimer’s disease. Life Sci. 2022;297:120470.
74. Zhu T, Guo J, Wu Y, et al. The mechanosensitive ion channel Piezo1 modulates the migration and immune response of microglia. iScience. 2023;26:105993.
75. Bouvier DS, Jones EV, Quesseveur G, et al. High resolution dissection of reactive glial nets in Alzheimer’s disease. Sci Rep. 2016;6:24544.
76. Kosoy R, Fullard JF, Zeng B, et al. Genetics of the human microglia regulome refines Alzheimer’s disease risk loci. Nat Genet. 2022;54:1145-54.
77. Liu H, Hu J, Zheng Q, et al. Piezo1 channels as force sensors in mechanical force-related chronic inflammation. Front Immunol. 2022;13:816149.
78. Yu D, Ahmed A, Jayasi J, Womac A, Sally O, Bae C. Inflammation condition sensitizes Piezo1 mechanosensitive channel in mouse cerebellum astrocyte. Front Cell Neurosci. 2023;17:1200946.
79. Ayata P, Badimon A, Strasburger HJ, et al. Epigenetic regulation of brain region-specific microglia clearance activity. Nat Neurosci. 2018;21:1049-60.
80. Khatri N, Thakur M, Pareek V, Kumar S, Sharma S, Datusalia AK. Oxidative stress: major threat in traumatic brain injury. CNS Neurol Disord Drug Targets. 2018;17:689-95.
81. Massaro M, Scoditti E, Carluccio MA, De Caterina R. Oxidative stress and vascular stiffness in hypertension: a renewed interest for antioxidant therapies?. Vascul Pharmacol. 2019;116:45-50.
82. Bai H, Yang B, Yu W, Xiao Y, Yu D, Zhang Q. Cathepsin B links oxidative stress to the activation of NLRP3 inflammasome. Exp Cell Res. 2018;362:180-7.
83. Hong Z, Tian Y, Yuan Y, et al. Enhanced oxidative stress is responsible for TRPV4-induced neurotoxicity. Front Cell Neurosci. 2016;10:232.
84. Bai JZ, Lipski J. Involvement of TRPV4 channels in Aβ40-induced hippocampal cell death and astrocytic Ca2+ signalling. Neurotoxicology. 2014;41:64-72.
85. Zhang X, Leng S, Liu X, et al. Ion channel Piezo1 activation aggravates the endothelial dysfunction under a high glucose environment. Cardiovasc Diabetol. 2024;23:150.
86. Velasco-Estevez M, Mampay M, Boutin H, et al. Infection augments expression of mechanosensing Piezo1 channels in amyloid plaque-reactive astrocytes. Front Aging Neurosci. 2018;10:332.
87. Wang B, Ke W, Wang K, et al. Mechanosensitive ion channel Piezo1 activated by matrix stiffness regulates oxidative stress-induced senescence and apoptosis in human intervertebral disc degeneration. Oxid Med Cell Longev. 2021;2021:8884922.
88. Chen J, Zhou Y, Liu S, Li C. Biomechanical signal communication in vascular smooth muscle cells. J Cell Commun Signal. 2020;14:357-76.
89. Herr I, Wilhelm D, Meyer E, Jeremias I, Angel P, Debatin KM. JNK/SAPK activity contributes to TRAIL-induced apoptosis. Cell Death Differ. 1999;6:130-5.
90. Waetzig V, Czeloth K, Hidding U, et al. c-Jun N-terminal kinases (JNKs) mediate pro-inflammatory actions of microglia. Glia. 2005;50:235-46.
91. Onyango IG, Bennett JP Jr, Tuttle JB. Endogenous oxidative stress in sporadic Alzheimer’s disease neuronal cybrids reduces viability by increasing apoptosis through pro-death signaling pathways and is mimicked by oxidant exposure of control cybrids. Neurobiol Dis. 2005;19:312-22.
92. Hsieh CJ, Kuo PL, Hsu YC, Huang YF, Tsai EM, Hsu YL. Arctigenin, a dietary phytoestrogen, induces apoptosis of estrogen receptor-negative breast cancer cells through the ROS/p38 MAPK pathway and epigenetic regulation. Free Radic Biol Med. 2014;67:159-70.
93. Cianciulli A, Porro C, Calvello R, Trotta T, Lofrumento DD, Panaro MA. Microglia mediated neuroinflammation: focus on PI3K modulation. Biomolecules. 2020;10:137.
94. Hirashima T, Hino N, Aoki K, Matsuda M. Stretching the limits of extracellular signal-related kinase (ERK) signaling - cell mechanosensing to ERK activation. Curr Opin Cell Biol. 2023;84:102217.
96. Chi S, Cui Y, Wang H, et al. Astrocytic Piezo1-mediated mechanotransduction determines adult neurogenesis and cognitive functions. Neuron. 2022;110:2984-99.e8.
97. Wang YY, Zhang H, Ma T, et al. Piezo1 mediates neuron oxygen-glucose deprivation/reoxygenation injury via Ca2+/calpain signaling. Biochem Biophys Res Commun. 2019;513:147-53.
98. Ji C, McCulloch CA. TRPV4 integrates matrix mechanosensing with Ca2+ signaling to regulate extracellular matrix remodeling. FEBS J. 2021;288:5867-87.
99. Shi M, Du F, Liu Y, et al. Glial cell-expressed mechanosensitive channel TRPV4 mediates infrasound-induced neuronal impairment. Acta neuropathol. 2013;126:725-39.
100. Ungvari Z, Wolin MS, Csiszar A. Mechanosensitive production of reactive oxygen species in endothelial and smooth muscle cells: role in microvascular remodeling?. Antioxid Redox Signal. 2006;8:1121-9.
101. Waters CM. Reactive oxygen species in mechanotransduction. Am J Physiol Lung Cell Mol Physiol. 2004;287:L484-5.
102. Simpson DSA, Oliver PL. ROS generation in microglia: understanding oxidative stress and inflammation in neurodegenerative disease. Antioxidants. 2020;9:743.
103. Fitzpatrick AW, Park ST, Zewail AH. Exceptional rigidity and biomechanics of amyloid revealed by 4D electron microscopy. Proc Natl Acad Sci U S A. 2013;110:10976-81.
104. Nassar R, Wong E, Gsponer J, Lamour G. Inverse correlation between amyloid stiffness and size. J Am Chem Soc. 2018;141:58-61.
105. Khan MI, Gilpin K, Hasan F, Mahmud KAHA, Adnan A. Effect of strain rate on single tau, dimerized tau and tau-microtubule interface: a molecular dynamics simulation study. Biomolecules. 2021;11:1308.
106. Hagestedt T, Lichtenberg B, Wille H, Mandelkow EM, Mandelkow E. Tau protein becomes long and stiff upon phosphorylation: correlation between paracrystalline structure and degree of phosphorylation. J Cell Biol. 1989;109:1643-51.
107. Zempel H, Dennissen FJA, Kumar Y, et al. Axodendritic sorting and pathological missorting of Tau are isoform-specific and determined by axon initial segment architecture. J Biol Chem. 2017;292:12192-207.
108. Lulevich V, Zimmer CC, Hong H, Jin L, Liu G. Single-cell mechanics provides a sensitive and quantitative means for probing amyloid-β peptide and neuronal cell interactions. Proc Natl Acad Sci U S A. 2010;107:13872-7.
109. Murphy MC, Jones DT, Jack CR Jr, et al. Regional brain stiffness changes across the Alzheimer’s disease spectrum. Neuroimage Clin. 2016;10:283-90.
110. Rivera-Rivera LA, Eisenmenger L, Cody KA, et al. Cerebrovascular stiffness and flow dynamics in the presence of amyloid and tau biomarkers. Alzheimers Dement. 2021;13:e12253.
111. Chakraborty R, Goswami C. Both heat-sensitive TRPV4 and cold-sensitive TRPM8 ion channels regulate microglial activity. Biochem Biophys Res Commun. 2022;611:132-9.
112. Redmon SN, Yarishkin O, Lakk M, et al. TRPV4 channels mediate the mechanoresponse in retinal microglia. Glia. 2021;69:1563-82.
113. Bai J, Lipski J. Differential expression of TRPM2 and TRPV4 channels and their potential role in oxidative stress-induced cell death in organotypic hippocampal culture. NeuroToxicology. 2010;31:204-14.
114. Sianati S, Schroeter L, Richardson J, Tay A, Lamandé SR, Poole K. Modulating the mechanical activation of TRPV4 at the cell-substrate interface. Front Bioeng Biotechnol. 2020;8:608951.
115. Du K, Dou Y, Chen K, Zhao Y. Activation of TRPV4 induces intraneuronal tau hyperphosphorylation via cholesterol accumulation. Exp Neurol. 2023;364:114392.
116. Chen M, Li X. Role of TRPV4 channel in vasodilation and neovascularization. Microcirculation. 2021;28:e12703.
117. Owens P, O’Brien E. Hypotension in patients with coronary disease: can profound hypotensive events cause myocardial ischaemic events?. Heart. 1999;82:477-81.
118. Pluta R, Ułamek M, Jabłoński M. Alzheimer’s mechanisms in ischemic brain degeneration. Anat Rec. 2009;292:1863-81.
119. Kalaria RN. Cerebrovascular degeneration is related to amyloid-beta protein deposition in Alzheimer’s disease. Ann N Y Acad Sci. 1997;826:263-71.
120. Yu ZH, Ji YC, Li K, et al. Stiffness of the extracellular matrix affects apoptosis of nucleus pulposus cells by regulating the cytoskeleton and activating the TRPV2 channel protein. Cell Signal. 2021;84:110005.
121. Doñate-Macián P, Enrich-Bengoa J, Dégano IR, Quintana DG, Perálvarez-Marín A. Trafficking of stretch-regulated TRPV2 and TRPV4 channels inferred through interactomics. Biomolecules. 2019;9:791.
123. Richardson J, Kotevski A, Poole K. From stretch to deflection: the importance of context in the activation of mammalian, mechanically activated ion channels. FEBS J. 2022;289:4447-69.
124. Bavi N, Richardson J, Heu C, Martinac B, Poole K. PIEZO1-mediated currents are modulated by substrate mechanics. ACS Nano. 2019;13:13545-59.
125. Pathak MM, Nourse JL, Tran T, et al. Stretch-activated ion channel Piezo1 directs lineage choice in human neural stem cells. Proc Natl Acad Sci U S A. 2014;111:16148-53.
126. Ellefsen KL, Holt JR, Chang AC, et al. Myosin-II mediated traction forces evoke localized Piezo1-dependent Ca2+ flickers. Commun Biol. 2019;2:298.
127. Li Y, Hu H, Butterworth MB, Tian JB, Zhu MX, O’Neil RG. Expression of a diverse array of Ca2+-activated K+ channels (SK1/3, IK1, BK) that functionally couple to the mechanosensitive TRPV4 channel in the collecting duct system of kidney. PLoS One. 2016;11:e0155006.
128. Sarkar S. Microglial ion channels: key players in non-cell autonomous neurodegeneration. Neurobiol Dis. 2022;174:105861.
129. Fukuda N, Toriuchi K, Mimoto R, et al. Hypothermia attenuates neurotoxic microglial activation via TRPV4. Neurochem Res. 2023;49:800-13.
130. Kamash P, Ding Y. Hypothermia promotes synaptic plasticity and protective effects in neurological diseases. Brain Circ. 2021;7:294-7.
131. Macaya A. Apoptosis in the nervous system. Rev Neurol. 1996;24:1356-60. Available from: https://europepmc.org/article/med/8974737. [Last accessed on 27 Apr 2025].
132. Sedding DG, Homann M, Seay Y, Tillmanns H, Preissner KT, Braun-Dullaeus RC. Calpain counteracts mechanosensitive apoptosis of vascular smooth muscle cells in vitro and in vivo. FASEB J. 2008;22:579-89.
133. Mahaman YAR, Huang F, Kessete Afewerky H, Maibouge TMS, Ghose B, Wang X. Involvement of calpain in the neuropathogenesis of Alzheimer’s disease. Med Res Rev. 2019;39:608-30.
134. Duszyc K, Gomez GA, Lagendijk AK, et al. Mechanotransduction activates RhoA in the neighbors of apoptotic epithelial cells to engage apical extrusion. Curr Biol. 2021;31:1326-36.e5.
135. Eisenhoffer GT, Loftus PD, Yoshigi M, et al. Crowding induces live cell extrusion to maintain homeostatic cell numbers in epithelia. Nature. 2012;484:546-9.
136. Abe J, Berk BC. Novel mechanisms of endothelial mechanotransduction. Arterioscler Thromb Vasc Biol. 2014;34:2378-86.
137. Kawaue T, Yow I, Pan Y, et al. Inhomogeneous mechanotransduction defines the spatial pattern of apoptosis-induced compensatory proliferation. Dev Cell. 2023;58:267-77.e5.
138. Xu X, Shen X, Wang J, et al. YAP prevents premature senescence of astrocytes and cognitive decline of Alzheimer’s disease through regulating CDK6 signaling. Aging Cell. 2021;20:e13465.
139. Tanaka H, Homma H, Fujita K, et al. YAP-dependent necrosis occurs in early stages of Alzheimer’s disease and regulates mouse model pathology. Nat Commun. 2020;11:507.
140. Rudzitis CN, Lakk M, Cullimore B, Krizaj D. TRPV4 contributes to stiffness-induced upregulation of YAP in the Trabecular Meshwork. Invest Ophthalmol Vis Sci. 2023;64:3452. Available from: https://iovs.arvojournals.org/article.aspx?articleid=2787756. [Last accessed on 27 Apr 2025].
141. Chen X, Sun FJ, Wei YJ, et al. Increased expression of transient receptor potential vanilloid 4 in cortical lesions of patients with focal cortical dysplasia. CNS Neurosci Ther. 2016;22:280-90.
142. Friedman D, Honig LS, Scarmeas N. Seizures and epilepsy in Alzheimer’s disease. CNS Neurosci Ther. 2012;18:285-94.
143. Geronzi U, Lotti F, Grosso S. Oxidative stress in epilepsy. Expert Rev Neurother. 2018;18:427-34.
144. Nass RD, Hampel KG, Elger CE, Surges R. Blood pressure in seizures and epilepsy. Front Neurol. 2019;10:501.
145. Wang Z, Zhou L, An D, et al. TRPV4-induced inflammatory response is involved in neuronal death in pilocarpine model of temporal lobe epilepsy in mice. Cell Death Dis. 2019;10:386.
146. An D, Qi X, Li K, et al. Blockage of TRPV4 downregulates the nuclear factor-kappa B signaling pathway to inhibit inflammatory responses and neuronal death in mice with pilocarpine-induced status epilepticus. Cell Mol Neurobiol. 2023;43:1283-300.
147. Means JC, Gerdes BC, Kaja S, et al. Caspase-3-dependent proteolytic cleavage of tau causes neurofibrillary tangles and results in cognitive impairment during normal aging. Neurochem Res. 2016;41:2278-88.
148. Glushakova OY, Glushakov AO, Borlongan CV, Valadka AB, Hayes RL, Glushakov AV. Role of caspase-3-mediated apoptosis in chronic caspase-3-cleaved tau accumulation and blood-brain barrier damage in the corpus callosum after traumatic brain injury in rats. J Neurotrauma. 2018;35:157-73.
149. Donnaloja F, Limonta E, Mancosu C, et al. Unravelling the mechanotransduction pathways in Alzheimer’s disease. J Biol Eng. 2023;17:22.
150. Niethammer P. Components and mechanisms of nuclear mechanotransduction. Ann Rev Cell Dev Biol. 2021;37:233-56.
151. Lomakin AJ, Cattin CJ, Cuvelier D, et al. The nucleus acts as a ruler tailoring cell responses to spatial constraints. Science. 2020;370:eaba2894.
152. Nava MM, Miroshnikova YA, Biggs LC, et al. Heterochromatin-driven nuclear softening protects the genome against mechanical stress-induced damage. Cell. 2020;181:800-17.e22.
153. Sun X, Eastman G, Shi Y, et al. Structural and functional damage to neuronal nuclei caused by extracellular tau oligomers. Alzheimers Dement. 2024;20:1656-70.
154. Pettus BJ, Chalfant CE, Hannun YA. Ceramide in apoptosis: an overview and current perspectives. Biochim Biophys Acta. 2002;1585:114-25.
155. Jazvinšćak Jembrek M, Hof PR, Šimić G. Ceramides in Alzheimer’s disease: key mediators of neuronal apoptosis induced by oxidative stress and Aβ accumulation. Oxid Med Cell Longev. 2015;2015:346783.
156. Chen CL, Lin CF, Chang WT, Huang WC, Teng CF, Lin YS. Ceramide induces p38 MAPK and JNK activation through a mechanism involving a thioredoxin-interacting protein-mediated pathway. Blood. 2008;111:4365-74.
157. Opferman JT, Kothari A. Anti-apoptotic BCL-2 family members in development. Cell Death Differ. 2018;25:37-45.
158. Ganesan V, Colombini M. Regulation of ceramide channels by Bcl-2 family proteins. FEBS Lett. 2010;584:2128-34.
159. Leiphart RJ, Chen D, Peredo AP, Loneker AE, Janmey PA. Mechanosensing at cellular interfaces. Langmuir. 2019;35:7509-19.
160. Thodeti CK, Matthews B, Ravi A, et al. TRPV4 channels mediate cyclic strain-induced endothelial cell reorientation through integrin-to-integrin signaling. Circ Res. 2009;104:1123-30.
161. Samakova A, Gazova A, Sabova N, Valaskova S, Jurikova M, Kyselovic J. The PI3k/Akt pathway is associated with angiogenesis, oxidative stress and survival of mesenchymal stem cells in pathophysiologic condition in ischemia. Physiol Res. 2019;68:S131-8.
162. Jie P, Hong Z, Tian Y, et al. Activation of transient receptor potential vanilloid 4 induces apoptosis in hippocampus through downregulating PI3K/Akt and upregulating p38 MAPK signaling pathways. Cell Death Dis. 2015;6:e1775.
163. Ong WY, Herr DR, Farooqui T, Ling EA, Farooqui AA. Role of sphingomyelinases in neurological disorders. Expert Opin Ther Targets 2015;19:1725-42.
164. Shi J, Hyman AJ, De Vecchis D, et al. Sphingomyelinase disables inactivation in endogenous PIEZO1 channels. Cell Rep. 2020;33:108225.
165. Shi J. How does fast-inactivation PIEZO1 channel sense constant mechanical forces in native endothelial cells?. Biophys J. 2023;122:160A.
166. Hunter OC. Mechanical cyclic strain induces ceramide generation in endothelial cells. 2009. Available from: http://d-scholarship.pitt.edu/8714/1/HunterOC_ETD2009.pdf. [Last accessed on 27 Apr 2025].
167. Czarny M, Liu J, Oh P, Schnitzer JE. Transient mechanoactivation of neutral sphingomyelinase in caveolae to generate ceramide. J Biol Chem. 2003;278:4424-30.
168. Razani B, Lisanti MP. Caveolins and caveolae: molecular and functional relationships. Exp Cell Res. 2001;271:36-44.
170. Niesman IR, Zemke N, Fridolfsson HN, et al. Caveolin isoform switching as a molecular, structural, and metabolic regulator of microglia. Mol Cell Neurosci. 2013;56:283-97.
171. Quest AFG, Lobos-González L, Nuñez S, et al. The caveolin-1 connection to cell death and survival. Curr Mol Med. 2013;13:266-81.
172. Gaudreault SB, Dea D, Poirier J. Increased caveolin-1 expression in Alzheimer’s disease brain. Neurobiol Aging. 2004;25:753-9.
173. Gupta A, Sharma A, Kumar A, Goyal R. Alteration in memory cognition due to activation of caveolin-1 and oxidative damage in a model of dementia of Alzheimer’s type. Indian J Pharmacol. 2019;51:173-80.
174. Wu Y, Lim YW, Parton RG. Caveolae and the oxidative stress response. Biochem Soc Trans. 2023;51:1377-85.
175. Bruno L, Karagil S, Mahmood A, Elbediwy A, Stolinski M, Mackenzie FE. Mechanosensing and the hippo pathway in microglia: a potential link to Alzheimer’s disease pathogenesis?. Cells. 2021;10:3144.
176. Qing J, Liu X, Wu Q, et al. Hippo/YAP pathway plays a critical role in effect of GDNF against Aβ-induced inflammation in microglial cells. DNA Cell Biol. 2020;39:1064-71.
177. Sahu MR, Mondal AC. The emerging role of Hippo signaling in neurodegeneration. J Neurosci Res. 2020;98:796-814.
178. Kim KS, Hwang HA, Chae SK, Ha H, Kwon KS. Upregulation of Daxx mediates apoptosis in response to oxidative stress. J Cell Biochem. 2005;96:330-8.
179. Cinar B, Collak FK, Lopez D, et al. MST1 is a multifunctional caspase-independent inhibitor of androgenic signaling. Cancer Res. 2011;71:4303-13.
180. Liu J, Wang J, Liu Y, et al. Liquid-liquid phase separation of DDR1 counteracts the Hippo pathway to orchestrate arterial stiffening. Circ Res. 2023;132:87-105.
181. Li D, Ji JX, Xu YT, et al. Inhibition of Lats1/p-YAP 1 pathway mitigates neuronal apoptosis and neurological deficits in a rat model of traumatic brain injury. CNS Neurosci Ther. 2018;24:906-16.
182. Rai SK, Savastano A, Singh P, Mukhopadhyay S, Zweckstetter M. Liquid-liquid phase separation of tau: from molecular biophysics to physiology and disease. Protein Sci. 2021;30:1294-314.
183. Ranganathan R, Li S, Sapozhnikov G, Wang S, Song YQ. Lower expression of BIN1’s neuronal isoform in vulnerable excitatory neurons increases risk in Alzheimer’s disease. J Alzheimers Dis Rep. 2025;9:25424823241296018.
184. Yu L, Chibnik LB, Srivastava GP, et al. Association of Brain DNA methylation in SORL1, ABCA7, HLA-DRB5, SLC24A4, and BIN1 with pathological diagnosis of Alzheimer disease. JAMA Neurol. 2015;72:15-24.
185. Peter BJ, Kent HM, Mills IG, et al. BAR domains as sensors of membrane curvature: the amphiphysin BAR structure. Science. 2004;303:495-9.
186. Dräger NM, Nachman E, Winterhoff M, et al. Bin1 directly remodels actin dynamics through its BAR domain. EMBO Rep. 2017;18:2051-66.
187. Povarova OI, Antifeeva IA, Fonin AV, Turoverov KK, Kuznetsova IM. The role of liquid-liquid phase separation in actin polymerization. Int J Mol Sci. 2023;24:3281.
189. Nadezhdina ES, Lomakin AJ, Shpilman AA, Chudinova EM, Ivanov PA. Microtubules govern stress granule mobility and dynamics. Biochim Biophys Acta. 2010;1803:361-71.
190. Li Y, Li L, Li B, et al. Mechanical stretching induces fibroblasts apoptosis through activating Piezo1 and then destroying actin cytoskeleton. Int J Med Sci. 2023;20:771-80.
191. Kommaddi RP, Das D, Karunakaran S, et al. Aβ mediates F-actin disassembly in dendritic spines leading to cognitive deficits in Alzheimer’s disease. J Neurosci. 2018;38:1085-99.
192. Samir P, Kesavardhana S, Patmore DM, et al. DDX3X acts as a live-or-die checkpoint in stressed cells by regulating NLRP3 inflammasome. Nature. 2019;573:590-4.
193. Sidibé H, Dubinski A, Vande Velde C. The multi-functional RNA-binding protein G3BP1 and its potential implication in neurodegenerative disease. J Neurochem. 2021;157:944-62.
194. Matsuki H, Takahashi M, Higuchi M, Makokha GN, Oie M, Fujii M. Both G3BP1 and G3BP2 contribute to stress granule formation. Genes Cells. 2013;18:135-46.
195. Somasekharan SP, Zhang F, Saxena N, et al. G3BP1-linked mRNA partitioning supports selective protein synthesis in response to oxidative stress. Nucleic Acids Res. 2020;48:6855-73.
196. Feng Z, Mao Z, Yang Z, Liu X, Nakamura F. The force-dependent filamin A-G3BP1 interaction regulates phase-separated stress granule formation. J Cell Sci. 2023;136:jcs260684.
197. Gottlieb PA. A tour de force: the discovery, properties, and function of piezo channels. Curr Top Membr. 2017;79:1-36.
198. Zhang H, Yang Z, Nakamura F. Importance of the filamin A-Sav1 interaction in organ size control: evidence from transgenic mice. Int J Dev Biol. 2023;67:27-37.
199. Wei SC, Fattet L, Tsai JH, et al. Matrix stiffness drives epithelial-mesenchymal transition and tumour metastasis through a TWIST1-G3BP2 mechanotransduction pathway. Nat Cell Biol. 2015;17:678-88.
200. Matrongolo MJ, Ang PS, Wu J, et al. Piezo1 agonist restores meningeal lymphatic vessels, drainage, and brain-CSF perfusion in craniosynostosis and aged mice. J Clin Invest. 2023;134:e171468.
201. Yang P, Mathieu C, Kolaitis RM, et al. G3BP1 is a tunable switch that triggers phase separation to assemble stress granules. Cell. 2020;181:325-45.e28.
202. Tauler J, Zudaire E, Liu H, Shih J, Mulshine JL. hnRNP A2/B1 modulates epithelial-mesenchymal transition in lung cancer cell lines. Cancer Res. 2010;70:7137-47.
Cite This Article

How to Cite
Download Citation
Export Citation File:
Type of Import
Tips on Downloading Citation
Citation Manager File Format
Type of Import
Direct Import: When the Direct Import option is selected (the default state), a dialogue box will give you the option to Save or Open the downloaded citation data. Choosing Open will either launch your citation manager or give you a choice of applications with which to use the metadata. The Save option saves the file locally for later use.
Indirect Import: When the Indirect Import option is selected, the metadata is displayed and may be copied and pasted as needed.
About This Article
Special Issue
Copyright
Data & Comments
Data
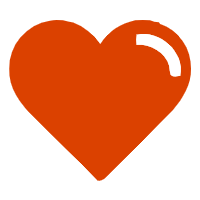
Comments
Comments must be written in English. Spam, offensive content, impersonation, and private information will not be permitted. If any comment is reported and identified as inappropriate content by OAE staff, the comment will be removed without notice. If you have any queries or need any help, please contact us at support@oaepublish.com.