The necessity for skeletal muscle contractile assays to assess treatment efficacy in DMD
Abstract
Body movement relies on skeletal muscles generating power to move limbs effectively. Power is defined as force multiplied by velocity: a muscle produces force at a specific velocity (the speed of muscle shortening) and this results in power. In diseases like Duchenne Muscular Dystrophy (DMD), the absence of dystrophin weakens muscles and impairs their shortening velocity, leading to decreased power and consequently, impaired movement. Additionally, the diaphragm and heart muscles are also affected in DMD, causing difficulty breathing and impaired cardiac function. Compromised cardiorespiratory function can ultimately lead to death. Given the complex etiology of DMD and the essential role of power in all affected muscles, it is crucial to assess potential treatments for their effectiveness in improving muscle function. This review focuses on fundamental physiological assays used to evaluate muscle function in skeletal and diaphragm muscles. Common assays include force-frequency, force-velocity, power, and eccentric protocols, which are conducted ex vivo, in situ, and in vivo in small rodents (such as mice and rats) and larger intermediate animal models such as the Golden Retriever Muscular Dystrophy dog. Existing data support the use of skeletal muscle contractile assays as objective tools for assessing the efficacy of treatments.
Keywords
INTRODUCTION
Duchenne Muscular Dystrophy (DMD) is a severe and fatal muscle wasting disease characterized by the absence of dystrophin due to mutations in the dystrophin gene that lead to the production of a non-functional protein. Because dystrophin is absent, the proteins in the dystrophin glycoprotein complex (DGC), also known as the Dystrophin Associated Protein Complex (DAPC), are also missing. The loss of the DGC/DAPC from the sarcolemma membrane disrupts mechanical stability and signaling, contributing to DMD[1,2]. In addition to heightened muscle vulnerability and poor regenerative capacity, which severely impair muscle function[2], individuals with DMD experience life-limiting complications, including disrupted lung function and progressive cardiomyopathy. As a result, DMD individuals often succumb to cardiac or respiratory failure[3,2]. A defining feature of DMD is the progressive loss of functional muscle tissue, which severely impacts contractile function[4]. While there is currently no known cure for DMD, several potential therapies, including micro-dystrophins and exon skipping using antisense oligonucleotides, are under investigation in clinical trials. Other promising treatments such as cell therapy, gene editing, and combination therapies have been extensively reviewed[1,2,5,6]. Regardless of the therapy, a critical goal of treatment is to improve contractile function.
In this review, we briefly describe contractile function, including the organization of skeletal muscle for force production and shortening (i.e., contraction), discuss the physiological methods used to assess contractile force and length change, highlight practical considerations for contractile assessments, and emphasize the importance of evaluating muscle function both preclinically and clinically to effectively assess a DMD treatment.
SKELETAL MUSCLE
Skeletal muscle organization
Motor units
Skeletal muscle is comprised of muscle fibers organized into motor units. A motor unit is defined as an alpha motor neuron and all the muscle fibers it innervates[7] [Figure 1]. The force produced by a muscle is dependent on the activation frequency or the rate at which action potentials are delivered to each of the motor units (i.e., rate coding), the number of motor units active at one time (i.e., recruitment), the number of fibers innervated by a single alpha motor neuron (i.e., the innervation ratio), and the contractile characteristics of the fibers in the motor units[7].
Figure 1. (A) Motor unit: an alpha motor neuron and all the fibers it innervates; (B) Anatomical structure of a skeletal muscle: muscle, fascicle, fiber, myofibril, sarcomere; (C) Sarcomere structure showing Z-lines, actin (thin) filaments and myosin (thick) filaments, and the M line (center of the sarcomere); (D) Actin thin filament segment showing the troponin-tropomyosin complex, which blocks the myosin heads from the actin-binding sites in the absence of Ca2+; a segment of a myosin thick filament, with myosin heads that interact with actin-binding sites; a cross-section of a myofibril showing actin thin filaments surrounding the myosin thick filaments.
Muscle fibers
Muscle fibers are composed of myofibrils, which contain the sarcomere, the functional unit of contraction. Sarcomeres are bounded by Z-lines. Attached to the Z-lines are the thin or actin filaments. To produce force and shorten the sarcomeres, the actin filaments are moved by thick myosin filaments [Figure 1]. Myosin filaments consist of myosin molecules, which are hexameric - composed of two myosin heavy chains and four light chains (not discussed). Each myosin heavy chain functions as a myosin motor, with heads that contain binding sites for Adenosine triphosphate (ATP). ATP is subsequently hydrolyzed to provide energy for the myosin head to physically move the actin filament. Thus, myosin heads exhibit ATPase activity. When the ATPase reaction is complete [Adenosine diphosphate (ADP) and inorganic phosphate, the hydrolysis products of ATP], the myosin head binds to the actin filament, pulling it toward the center of the sarcomere, known as the M line (i.e., sarcomere shortening; Figure 1). Sarcomeres are arranged in series in the myofibril, like the cars of a train linked together. When all the sarcomeres contract, the myofibril shortens. Myofibrils are aligned in parallel in a muscle fiber, so when the myofibrils shorten, the muscle fiber itself also shortens. All the fibers within a motor unit contract simultaneously (All-or-None Principle). When multiple motor units within a muscle contract simultaneously, the muscle produces force and shortens[8].
Contractile regulation
Muscle contraction is regulated by the thin filament because the regulatory proteins are located on the actin filament. In brief, when the myosin binding sites on actin are unavailable, myosin cannot enter the strong-binding state. However, when these binding sites become available, myosin can enter the strong-binding state, and contraction can occur, provided that both calcium and ATP are present. Calcium is essential for binding to a protein called Troponin C (specific for calcium) on the actin filament. When calcium binds to Troponin C, it causes the troponin-tropomyosin complex, which normally blocks the myosin binding sites on actin, to be removed [Figure 1]. This allows myosin to bind to actin and use the energy from ATP hydrolysis to move the actin filament, generating force and shortening the muscle. When calcium is absent, contraction stops, i.e., the muscle relaxes[8]. Although the main regulatory mechanism is the availability of myosin binding sites on actin in response to Ca2+ binding to troponin C (i.e., thin-filament regulation), a thick-filament-based regulatory mechanism has also been proposed, which is thought to match the load experienced by the sarcomeres with the addition of myosin heads, independent of thin-filament regulation[9].
In a healthy muscle, assuming adequate oxygen and substrate availability, fiber ATP concentration is near constant below exhaustion levels of contraction because ATP production is well supported by intermediary metabolism[10]. The availability of calcium to bind to actin depends on action potentials from the alpha motor neuron arriving at the neuromuscular junction. The release of acetylcholine from the axon terminal generates an action potential along the muscle fiber’s sarcolemma. This action potential then travels through the T-tubules, triggering the release of calcium from the sarcoplasmic reticulum. The calcium then diffuses through the myoplasm to bind to Troponin C. When the action potentials cease, calcium is released from Troponin C and resequestered into the sarcoplasmic reticulum, and contraction stops, leading to relaxation. Therefore, calcium is tightly regulated in a healthy muscle to ensure that contraction only occurs in response to an action potential. This regulation is especially important for the diaphragm, ensuring rhythmic contraction-relaxation for breathing, but also critical for body movements[8].
Dystrophic muscle
In the 1970s and 1980s, the Neural hypothesis proposed that muscle changes in DMD were a result of abnormal trophic influences from motor neurons (e.g., in DMD individuals[11] and dystrophic mice[12]). However, the main influence identified for the decline in muscle function in DMD was a reduction in the number of functional motor units[11]. To the authors’ knowledge, the Neural hypothesis has not been extensively pursued. Current studies are focusing on the role of dystrophin in brain development and its potential implications in Duchenne and Becker muscular dystrophies[13]. The interaction between the axon of the motor neuron and each fiber it innervates occurs at the neuromuscular junction (NMJ). Defects in the NMJ of DMD muscle fibers have been reported[14,15], possibly due to the potential role of dystrophin in satellite cell function[16]. In addition to the dysfunction at the NMJ, the brunt of DMD appears to be most evident in the muscle fibers, and therefore in DMD muscle.
At the muscle fiber level, the absence of dystrophin and the DGC results in poor muscle function. When muscle function is compromised, muscle power is compromised, and therefore body movement is compromised. Several mechanistic hypotheses have been proposed to explain the progressive and debilitating dystrophic processes that ultimately affect muscle function, including dysfunctional contractile regulation at the fiber level (e.g., abnormal calcium handling[17] and metabolic dysfunction[18]), impaired muscle regeneration, and the loss of functional muscle tissue, which is replaced by fatty and fibrotic infiltration, among others[4]. Thus, there are numerous mechanisms thought to contribute to the pathogenesis of DMD, and each represents a potential target for therapy[1]. For example, microdystrophins can be delivered with Adeno-associated Virus (AAV) vectors to replace the absent full-length dystrophin. Specific biochemical and molecular assays (e.g., Western blot, immunostaining[19]) can confirm the presence of the microdystrophin protein. However, the most reliable assessment of treatment efficacy is the overall benefit to muscle function.
Movement
Skeletal muscles are arranged in agonist and antagonist pairs on either side of a joint, such as the ankle. To ambulate, movement at the ankle joint requires the rhythmic contraction of the agonists (e.g., plantar flexors) paired with the relaxation of the antagonists (e.g., dorsiflexors), and then this sequence reverses to enable walking. These coordinated movements at joints facilitate body movement[20]. Broadly, for any movement to occur, the agonist muscles involved must produce force and shorten while the antagonists relax. When either the agonist or antagonist muscles contract, they generate force and shorten; the product of force and shortening speed is referred to as power[21]. Therefore, rhythmic muscle power production in agonists and antagonists is essential to movement[20]. The interaction between agonists and antagonists highlights the importance of measuring the function of both, as imbalances in the forces produced across a joint can lead to debilitating contractures, as seen in DMD. One reason for such imbalances could be true hypertrophy (i.e., increased muscle mass) that may occur early in disease development, or pseudohypertrophy (i.e., the deposition of fat and connective tissue) that follows. Both types of muscle enlargement are commonly observed in the calves of boys with DMD and can be detected through ultrasonography and magnetic resonance imaging (MRI) imaging. The imbalance between stronger ankle extensors and weaker ankle flexors can lead to equinus contracture, which results in toe walking among individuals with DMD[22]. For additional discussion on postural instability and contractures in individuals with DMD and DMD animal models, see Kornegay et al., 2012[22].
Individuals with DMD experience difficulty walking[23], adapt their gait patterns to maintain support, propulsion, and balance[24], and gradually lose the ability to ambulate[4,25,26]. To assess the loss of muscle function related to ambulation, several timed functional assays have been developed. These tests are designed to measure the time it takes for individuals with DMD to complete typical daily activities. For example, the 6-Minute Walk Test (6MWT) is often used to assess clinical status in individuals with DMD[27], including the evaluation of treatment efficacy[28]. Other timed functional assays include the North Star Ambulatory Assessment (NSAA), time to stand velocity (TTSTAND), time to run 10 m (TTRW), and time to climb four stairs (TTCLIMB)[28,29]. Data from these tests, collected over time, can provide an indirect measure of disease progression in an individual, as longer completion times may indicate worsening function, while improvements may suggest a positive treatment response.
Eccentric contractions
The absence of dystrophin in skeletal muscle is widely recognized to disrupt the mechanical integrity of dystrophic muscle fibers, making them more susceptive to injury from lengthening or eccentric contractions[30]. This relationship has been demonstrated in dystrophic animal models, such as the mdx mouse[31] and Golder Retriever Muscular Dystrophy (GRMD) dog[32]. Based on these and numerous other published findings in dystrophic animal models, and given the ethical limitations to directly test the response of DMD muscles, it can be logically inferred that DMD muscles are also vulnerable to injury from eccentric contractions.
Eccentric contractions occur during daily activities such as level walking, uphill/downhill walking, and ascending/descending stairs[33]. The loss of ambulation in boys with DMD is positively correlated with a decline in muscle quality, as measured by MRI, and with decreased step activity. Thus, step activity serves as a reliable predictor of ambulation loss in DMD boys[26]. A decrease in step activity may be due to injuries caused by eccentric contractions. Musculoskeletal simulations suggest that, depending on the type of movement, individual muscles experience varying degrees of eccentric contraction, which could lead to selective degeneration in the dystrophic muscles of the lower limb[34]. For example, simulations of healthy individuals walking downhill revealed greater eccentric contraction in the lower limb muscles, while uphill walking showed similar contraction levels to level walking[33]. These findings support the recommendation for individuals with DMD to avoid activities that emphasize eccentric contractions (such as walking downstairs or walking downhill). To counteract ambulation loss, exercise may be beneficial. Boys with DMD have shown positive responses to a moderate resistance training program, which improved muscle strength and functional ability in activities like descending stairs[25]. A typical method for evaluating the loss of strength in dystrophin-deficient muscles is through eccentric contraction protocols (see below).
ASSESSMENT OF MUSCLE FUNCTION BY CONTRACTILE ASSAYS
Although muscles are composed of motor units, it is difficult to activate individual or multiple motor units in whole muscle preparations, either in the lab or in the anesthetized animals. In physiological assays, the muscle is electrically activated, including all motor units; ideally, this means the entire muscle is activated. Electrical stimulation mimics action potentials generated by alpha motor neurons, and this is achieved using an electrical stimulator[35].
Types of contractile assessments
Typical assays include ex vivo (also known as in vitro[35]), in situ, and in vivo approaches, used in small rodents such as mice and rats, and larger intermediate animal models like the GRMD dog[36,37].
Ex vivo (in vitro)
This approach involves an isolated mouse skeletal muscle preparation (for details, see[35]). Briefly, muscles such as the extensor digitorum longus (EDL), soleus, or diaphragm are isolated from deeply anesthetized mice. The muscle is then mounted in a temperature-controlled organ bath with an appropriate salt solution to mimic the animal’s physiological conditions. Electrical stimulation is delivered via closely flanking platinum electrodes to elicit muscle contractions. Static or dynamic force is measured using a transducer [Figure 2][35].
Figure 2. Contractile assay methods. (A) Ex vivo (e.g., isolated mouse soleus); (B) In situ (e.g., mouse gastrocnemius); (C) In vivo (e.g., mouse plantar flexors) contractile assessment methods. For In vivo, the arrow indicates the direction of force; (D) Torque is the product of force and the moment arm (MA), where MA is the distance from the axis of rotation to the point where the force is applied. Torque is expressed in millinewtons multiplied by meters (mN·m).
In situ
This approach uses an intact muscle preparation, preserving both innervation and vasculature. Typically, muscles such as the tibialis anterior (TA), gastrocnemius, plantaris, or soleus are evaluated in animals (mouse, rat, or dog). The overlying skin is incised, and the muscle is kept moist and warmed using paraffin or a heat lamp. A suture is tied to the distal tendon and to a transducer, after which the tendon is severed distal to the suture knot. Electrical stimulation is applied via electrodes to induce muscle contractions through the appropriate nerve (e.g., the radial nerve for the extensor carpi ulnaris[38]. Static or dynamic force is measured using a transducer [Figure 2]).
In vivo
This approach assesses the contractile function of muscle groups in the forelimbs or hindlimbs of intact animals (mouse[19], rat, dog[39,40]). In this method, muscle groups are electrically stimulated using needle electrodes, activating contractions via the appropriate nerve (e.g., the tibial nerve for plantar flexors[19]). Isometric (static) or dynamic force can then be measured with a force transducer. In in vivo analysis, torque is often calculated. Torque is the product of a force and its moment arm (MA), where MA is the distance from the axis of rotation to the applied force [Figure 2]. Torque is commonly expressed relative to animal body mass (e.g., mN•m/g for mice[19]).
Eccentric
A common method for evaluating the strength loss in dystrophin-deficient muscle in the DMD field, or assessing the potential for a given therapy to reduce force loss is the use of eccentric contraction protocols ex vivo, in situ, or in vivo. Typically, in contractile assessments, the muscle group (in vivo) or individual muscle (ex vivo, in situ) is electrically stimulated to induce an isometric tetanus. During the plateau phase of tetanus, the muscle group or muscle is stretched at a specific shortening speed to a predetermined percentage of its optimal length (Lo). Eccentric contractions can be repeated multiple times with a set rest period between each stretch. The loss of isometric force is then plotted as a function of the number of stretches. The extent of force loss is dependent on several extrinsic and intrinsic factors. For a comprehensive review of eccentric contractions, refer to Kiriaev et al., 2023[41], and consult the references in Table 1, which assessed eccentric contractions in mdx mice or dystrophin-deficient dogs.
Representative references (2019-2024) in DMD research that used Ex vivo, In situ or In vivo contractile assays on muscles from dystrophic mice and dogs
Authors | Assay | Model | Age | Muscle(s) | Contractile Properties | Treatment/ manipulation | Significant change in muscle performance |
Maxwell et al.[42] | Ex vivo | Male mdx mice | 1-4 mo | Dia | Isom F-F | NAC | N |
Russell et al.[43] | Ex vivo | Male mdx mice | 10-16 wks | EDL, Sol, Lumb | Isom Tet & Ecc | EDG5506 | Y |
Hamm et al.[44] | Ex vivo | Male mdx mice | 7-61 wks | Sol & Dia | Isom F-F, F-V, Power, Ecc | Ex & µDys | Y |
García-Castañeda et al.[45] | Ex vivo | Male mdx-Orai1-KO mice | 13-16 wks | EDL and Sol | Isom Fat and Ecc | Orai1 | Y |
Hamm et al.[19] | Ex vivo | Male mdx mice | 7-28 wks | EDL and Dia | Isom T-F, T-V, Power, Ecc | Ex & µDys | Y |
Lindsay et al.[46] | Ex vivo | Male mdx mice | 7 mo | EDL | Isom F-F | Isom RT | Y |
Addinsall et al.[47] | Ex vivo | Male mdx mice | 4-7 wks | EDL & Sol | Isom F-F & Fat | ADAMTS-5 antibody | EDL-Y; Sol-N |
Ramos et al.[48] | Ex vivo | Male mdx4cv mice | 2-25 wks | Dia | Isom Tet & Ecc | Several µDys | Y |
Monceau et al.[49] | In situ | Female D2-mdx mice | 5-6 mo | TA | Isom Tet | Ex & Exon skipping (AAV-U7 snRNA) | N |
Ramos et al.[48] | In situ | Male mdx4cv mice | 2-25 wks | Gastroc | Isom Tet & Ecc | Several µDys | Y |
Russell et al.[43] | In situ | Male mdx mice | 8-14 wks | TA | Isom Tet & Ecc | EDG5506 | Y |
Kodippili et al.[38] | In situ | Male dystrophin-/- dogs | 5-36 mo | ECU | Tw/Tet & Ecc | SERCA overexpression | Y |
Hakim et al.[50] | In situ | Male dystrophin-/- dogs | 36 mo | ECU | Tw/Tet & Ecc | N/A; compare to normal dogs | Y |
Lindsay et al.[46] | In vivo | Male mdx mice | 6 mo | Ant Crural muscles | Isom T-F & Ecc | Isom RT | Y |
Hamm et al.[44] | In vivo | Male mdx mice | 7-54 wks | Plantar flexors | Isom T-F, T-V, Power, Ecc | Ex & µDys | Y |
Hamm et al.[19] | In vivo | Male mdx mice | 7-28 wks | Plantar flexors | Isom T-F, T-V, Power, Ecc | Ex & µDys | Y |
Birch et al.[37] | In vivo | Male and Female GRMD | 3 mo | TTJ Flex/Ext | TTJ Flex/Ext torque | µDys | Flx-Y; Ext-N |
Riddel et al.[51] | In vivo | Male DE50-MD | 3-18 mo | TTJ Flex/Ext | Isom T-F & Ecc | N/A; compare to normal dogs | Y |
Twitch kinetics
Typical analysis of skeletal muscle contractile responses includes the characteristics of the twitch. Twitch contractile responses are those in response to a single electrical activation pulse. Analysis can reveal potential differences between wild type and mdx skeletal muscles for the peak force produced, and alterations in the kinetics such as the time to peak force and half-relaxation time, among others. These characteristics are described for ex vivo contractile assessments in Sperringer and Grange, 2016[35], but are equally relevant to in situ or in vivo contractile assessments.
Selection of assessment approach
The choice of assessment approach(es) is determined by the specific needs of the investigator(s). All approaches can distinguish differences in skeletal muscle performance between control and mdx mice, or between control and dystrophin-deficient dogs. In general, the in vivo approach is suitable for tracking the same animals over time without the need for sacrifice until the study concludes (advantage: time-course data; intact internal milieu; disadvantage: only groups of muscles assessed). The in situ approach is appropriate when evaluating a specific muscle (e.g., the TA or gastrocnemius) rather than a group of muscles (e.g., plantar flexors), with the blood supply and nervous system remaining intact (advantage: single muscle assessed; intact internal milieu; disadvantage: animal must be sacrificed). The ex vivo approach is suited for evaluating the contractile properties of a specific muscle, excluding the potential influence of the internal milieu (advantage: single muscle assessed; disadvantage: may not fully represent in vivo or in situ function). For additional guidance, please see Practical considerations for assessing muscle function, General Considerations below.
Muscle contractile performance
Types of muscle contraction
Muscle contractions can be broadly described as either static or dynamic. A static contraction occurs when the muscle length remains unchanged (i.e., isometric contraction). Assays to assess static muscle contractile performance include the isometric twitch, isometric tetanus, the isometric force-frequency relationship, and fatigue test. Descriptions and examples of ex vivo contractile profiles for the isometric twitch, maximal tetanus, force-frequency relationship, and fatigue response are provided in[35].
A dynamic contraction involves the muscle generating force while shortening (i.e., concentric contraction) or lengthening (i.e., eccentric contraction). Assays to evaluate dynamic muscle contractile performance include force-velocity and eccentric injury contractions. Descriptions and examples of contractile profiles for the force-velocity relationship, determination of Vmax (i.e., maximal muscle shortening velocity), and power calculations can be found in[35], and for eccentric contractions[32,41].
Both static and dynamic assays can be conducted in ex vivo, in situ and in vivo experimental models [Figure 2]. Additionally, a novel method designed to mimic human gait using isolated soleus muscles ex vivo has been reported[51].
Figure 3 illustrates examples of in vivo mouse isometric torque contractions (profiles), the torque-frequency relationship, the torque-velocity relationship, and power output. Table 1 provides recent references on various contractile assessment methods used in dystrophic mice and dystrophin-null and GRMD dogs.
Figure 3. In vivo contractile responses of mouse plantar flexors. (A) Torque contractile profiles at individual electrical stimulation frequencies. Note that the response to a single stimulation (the twitch) is presented separately; (B) Torque-Frequency relationship, showing the maximum torque at each electrical stimulation frequency; (C) Torque-Velocity relationship, depicting normalized torque (each torque value divided by the mouse’s body mass) at specific shortening velocities (in degrees per second); (D) Normalized Power derived from the torque-velocity relationship. Power is calculated as the product of force and the velocity, expressed in milliwatts/gram body mass. Data from wild-type (WT) and mdx mice aged 33 weeks (for in vivo methods, see Hamm et al., 2023[43]).
Practical considerations for assessing muscle function
There are numerous potential pitfalls in obtaining good contractile data from any of the available contractile assessment methods. Experts in specific methods will have a detailed Standard Operating Procedure (SOP) to obtain consistent contractile data. Below are some key suggestions; note that the list is not exhaustive.
General considerations
a. Determine the contractile assessment method that best suits the goals of the study.
b. Collaborate with an expert familiar with the chosen contractile assessment method either to conduct the assays or to train lab personnel, as well as guide data analysis and interpretation.
c. Understand the equipment specifications and ensure appropriate settings [consult the manual(s)].
d. Compare contractile values to those reported in the literature, especially for control animals. Ensure the contractile values are consistent with those for the same animal genotype, age, mass, and sex. If the values deviate by more than 5%-10% from those reported in the literature, troubleshoot the methods.
Common steps for all contractile assessment types
a. Ensure all equipment is properly connected, with all connections securely fastened.
b. Use the correct transducer range to capture the expected range of muscle contractile values.
c. Verify that the settings on the electrical stimulator are correct.
d. Use the correct electrodes (typically platinum), ensuring they are the appropriate length for the assessment.
e. Set and maintain the appropriate muscle resting length throughout the experiment for data collection.
f. Test different voltages or currents as needed to produce the maximum twitch or tetanus to determine resting length before collecting experimental data.
Ex vivo considerations
a. Ensure the animal is deeply anesthetized during muscle dissection.
b. Handle the muscle with care during dissection and when positioning it in the bath and attaching it to the transducer. For example, an EDL muscle from a 25 g mouse has a length of ~12-14 mm and a mass of ~12-14 mg. Even minor handling errors can impair or completely prevent its contractile ability.
c. Ensure proper vertical alignment between the muscle and the transducer.
d. Ensure that the suture used to attach the muscle to the transducer is tightly knotted and does not slip during contractions, either at the tendon or where it connects to the transducer.
e. Ensure the platinum electrodes are closely flanking the muscle (within 2-5 mm).
In situ considerations
a. Ensure the animal is deeply anesthetized.
b. Maintain the animal and muscle at the appropriate temperature.
c. Ensure good mechanical alignment between the muscle and the transducer, and ensure the animal remains stable (i.e., does not move during data collection).
d. Ensure the suture or other material used to connect the muscle to the transducer is knotted tightly and not slipping during contractions.
e. Ensure the stimulating electrodes are secure in their position prior to and during the contractions; electrode movement can affect the magnitude of the contractile response.
f. Replace electrodes regularly (after 6-10 uses).
In vivo considerations
a. Ensure the animal is deeply anesthetized.
b. Keep the animal warm throughout the procedure.
c. Ensure good mechanical alignment between the animal and equipment.
d. Position the animal’s foot correctly on the foot pedal and secure it properly.
e. Ensure the knee is stabilized.
f. Ensure the electrodes are secure in their position prior to and during the contractions; electrode movement can affect the magnitude of the contractile response.
g. Replace electrodes regularly (after 6-10 uses).
Primary vs. secondary endpoints; other outcome measures
a. The goal of this review is to encourage the use of direct measures as primary endpoints for assessing skeletal muscle function, especially in preclinical models of DMD (e.g.,[19,44]).
b. In preclinical models of DMD (e.g., mdx mice, GRMD), additional outcomes or secondary endpoints such as gait, joint angles, ultrasound, MRI, and respiratory testing, among others, have proven valuable for providing a comprehensive assessment of the disease progression (e.g.,[22,51]).
c. In clinical trials, the direct measurement of skeletal muscle contractile function is more difficult, making secondary endpoints essential. For a recent discussion on the challenges of outcome measures in clinical trials for DMD, see Benemei et al., 2024[53].
CONCLUSION
Skeletal muscles produce force and shorten to enable breathing (e.g., diaphragm) and movement (e.g., walking). Skeletal muscle power is the product of force and the speed of muscle shortening. DMD muscles produce less power due to reduced force generation and slower shortening speeds, leading to significant impairments in breathing and movement. Several hypotheses have been proposed to explain the loss of muscle function in DMD, and several potential therapeutic approaches are being explored to rescue muscle function, force, and power. The most reliable measure of a treatment’s effectiveness in preclinical studies is the assessment of skeletal muscle contractile performance. Several studies [Table 1] have used various animal models and contractile assessment methods to evaluate potential improvements in muscle function. These studies support the use of skeletal muscle contractile assays to objectively assess treatment efficacy. Researchers in the DMD field are strongly encouraged to incorporate muscle function assessments in their preclinical studies, either through collaboration with experts in the chosen assessment method or by using well-trained and experienced personnel. If improving muscle function is a primary goal of preclinical treatments for DMD, it is essential to include muscle function assessments in the study design. This approach is equally important for clinical studies[53].
DECLARATIONS
Authors’ contributions
Made substantial contributions to the conception and content of the review and interpretation: Yuan C, Sweeten A, Grange RW
Figures were partially created in BioRender 2025(https://BioRender.com/o63u087): Yuan C
Availability of data and materials
Not applicable.
Financial support and sponsorship
None.
Conflicts of interest
All authors declared that there are no conflicts of interest.
Ethical approval and consent to participate
Not applicable.
Consent for publication
Not applicable.
Copyright
© The Author(s) 2025.
REFERENCES
1. Roberts TC, Wood MJA, Davies KE. Therapeutic approaches for Duchenne muscular dystrophy. Nat Rev Drug Discov. 2023;22:917-34.
2. Duan D, Goemans N, Takeda S, Mercuri E, Aartsma-Rus A. Duchenne muscular dystrophy. Nat Rev Dis Primers. 2021;7:13.
3. Birnkrant DJ, Bello L, Butterfield RJ, et al. Cardiorespiratory management of Duchenne muscular dystrophy: emerging therapies, neuromuscular genetics, and new clinical challenges. Lancet Respir Med. 2022;10:403-20.
4. Evans WJ, Hellerstein M, Butterfield RJ, et al. Reductions in functional muscle mass and ability to ambulate in Duchenne muscular dystrophy from ages 4 to 24 years. J Physiol. 2024;602:4929-39.
5. Himič V, Davies KE. Evaluating the potential of novel genetic approaches for the treatment of Duchenne muscular dystrophy. Eur J Hum Genet. 2021;29:1369-76.
6. Verhaart IEC, Aartsma-Rus A. Therapeutic developments for Duchenne muscular dystrophy. Nat Rev Neurol. 2019;15:373-86.
7. Duchateau J, Enoka RM. Distribution of motor unit properties across human muscles. J Appl Physiol. 2022;132:1-13.
8. Mukund K, Subramaniam S. Skeletal muscle: A review of molecular structure and function, in health and disease. Wiley Interdiscip Rev Syst Biol Med. 2020;12:e1462.
9. Irving M. Regulation of contraction by the thick filaments in skeletal muscle. Biophys J. 2017;113:2579-94.
10. Glancy B, Balaban RS. Energy metabolism design of the striated muscle cell. Physiol Rev. 2021;101:1561-607.
11. Sica RE, McComas AJ. The neural hypothesis of muscular dystrophy-a review of recent experimental evidence with particular reference to the Duchenne form. Can J Neurol Sci. 1978;5:189-97.
12. Bateson DS, Parry DJ. Motor units in a fast-twitch muscle of normal and dystrophic mice. J Physiol. 1983;345:515-23.
13. Fujimoto T, Mori M, Tonosaki M, et al. Characterization of dystrophin Dp71 expression and interaction partners in embryonic brain development: implications for duchenne/becker muscular dystrophy. Mol Neurobiol. ;2025:1-17.
14. Ng SY, Ljubicic V. Recent insights into neuromuscular junction biology in Duchenne muscular dystrophy: impacts, challenges, and opportunities. EBioMedicine. 2020;61:103032.
15. Lovering RM, Iyer SR, Edwards B, Davies KE. Alterations of neuromuscular junctions in Duchenne muscular dystrophy. Neurosci Lett. 2020;737:135304.
16. Ganassi M, Zammit PS. Involvement of muscle satellite cell dysfunction in neuromuscular disorders: expanding the portfolio of satellite cell-opathies. Eur J Transl Myol. 2022:32.
17. Mareedu S, Million ED, Duan D, Babu GJ. Abnormal calcium handling in Duchenne muscular dystrophy: mechanisms and potential therapies. Front Physiol. 2021;12:647010.
18. Gaglianone RB, Santos AT, Bloise FF, et al. Reduced mitochondrial respiration and increased calcium deposits in the EDL muscle, but not in soleus, from 12-week-old dystrophic mdx mice. Sci Rep. 2019;9:1986.
19. Hamm SE, Fathalikhani DD, Bukovec KE, et al. Voluntary wheel running complements microdystrophin gene therapy to improve muscle function in mdx mice. Mol Ther Methods Clin Dev. 2021;21:144-60.
20. Winter D. Biomechanics and motor control of human movement. 4th ed. John Wiley and Sons; 2009. p. 384.
21. Fitts RH, McDonald KS, Schluter JM. The determinants of skeletal muscle force and power: their adaptability with changes in activity pattern. J Biomech. 1991;24:111-22.
22. Kornegay JN, Childers MK, Bogan DJ, et al. The paradox of muscle hypertrophy in muscular dystrophy. Phys Med Rehabil Clin N Am. 2012;23:149-72.
23. Ramli AA, Liu X, Berndt K, et al. Gait characterization in Duchenne muscular dystrophy (DMD) using a single-sensor accelerometer: classical machine learning and deep learning approaches. Sensors. 2024;24:1123.
24. Gaudreault N, Gravel D, Nadeau S, Houde S, Gagnon D. Gait patterns comparison of children with Duchenne muscular dystrophy to those of control subjects considering the effect of gait velocity. Gait Posture. 2010;32:342-7.
25. Lott DJ, Taivassalo T, Senesac CR, et al. Walking activity in a large cohort of boys with Duchenne muscular dystrophy. Muscle Nerve. 2021;63:192-8.
26. Nair KS, Lott DJ, Forbes SC, et al. Step activity monitoring in boys with Duchenne muscular dystrophy and its correlation with magnetic resonance measures and functional performance. J Neuromuscul Dis. 2022;9:423-36.
27. McDonald CM, Henricson EK, Abresch RT, et al. PTC124-GD-007-DMD study group. The 6-minute walk test and other endpoints in Duchenne muscular dystrophy: longitudinal natural history observations over 48 weeks from a multicenter study. Muscle Nerve. 2013;48:343-56.
28. Pascual-Morena C, Lucerón-Lucas-Torres M, Martínez-García I, Rodríguez-Gutiérrez E, Patiño-Cardona S, Sequí-Domínguez I. Efficacy and safety of vamorolone in Duchenne muscular dystrophy: a systematic review. Paediatr Drugs. 2024;26:695-707.
29. Muntoni F, Signorovitch J, Sajeev G, et al. PRO-DMD-01 study investigators; Association Française contre les Myopathies; UK NorthStar Clinical Network; ImagingDMD investigators; cTAP. Meaningful changes in motor function in Duchenne muscular dystrophy (DMD): a multi-center study. PLoS One. 2024;19:e0304984.
30. Blaauw B, Agatea L, Toniolo L, et al. Eccentric contractions lead to myofibrillar dysfunction in muscular dystrophy. J Appl Physiol. 2010;108:105-11.
31. Baumann CW, Ingalls CP, Lowe DA. Mechanisms of weakness in Mdx muscle following in vivo eccentric contractions. J Muscle Res Cell Motil. 2022;43:63-72.
32. Tegeler CJ, Grange RW, Bogan DJ, et al. Eccentric contractions induce rapid isometric torque drop in dystrophin-deficient dogs. Muscle Nerve. 2010;42:130-2.
33. Hu X, Pickle NT, Grabowski AM, Silverman AK, Blemker SS. Muscle eccentric contractions increase in downhill and high-grade uphill walking. Front Bioeng Biotechnol. 2020;8:573666.
34. Hu X, Blemker SS. Musculoskeletal simulation can help explain selective muscle degeneration in Duchenne muscular dystrophy. Muscle Nerve. 2015;52:174-82.
35. Sperringer JE, Grange RW. In vitro assays to determine skeletal muscle physiologic function. In: Kyba M, editor. Skeletal muscle regeneration in the mouse. New York: Springer; 2016. pp. 271-91.
36. Kornegay JN, Bogan JR, Bogan DJ, et al. Canine models of Duchenne muscular dystrophy and their use in therapeutic strategies. Mamm Genome. 2012;23:85-108.
37. Birch SM, Lawlor MW, Conlon TJ, et al. Assessment of systemic AAV-microdystrophin gene therapy in the GRMD model of Duchenne muscular dystrophy. Sci Transl Med. 2023;15:eabo1815.
38. Kodippili K, Hakim CH, Burke MJ, et al. SERCA2a overexpression improves muscle function in a canine Duchenne muscular dystrophy model. Mol Ther Methods Clin Dev. 2024;32:101268.
39. Childers MK, Grange RW, Kornegay JN. In vivo canine muscle function assay. J Vis Exp. ;2011:2623.
40. Childers MK, Okamura CS, Bogan DJ, et al. Eccentric contraction injury in dystrophic canine muscle. Arch Phys Med Rehabil. 2002;83:1572-78.
41. Kiriaev L, Baumann CW, Lindsay A. Eccentric contraction-induced strength loss in dystrophin-deficient muscle: preparations, protocols, and mechanisms. J Gen Physiol. 2023:155.
42. Maxwell MN, Marullo AL, Valverde-Pérez E, Slyne AD, Murphy BT, O’Halloran KD. Chronic N-acetyl cysteine treatment does not improve respiratory system performance in the mdx mouse model of Duchenne muscular dystrophy. Exp Physiol. 2024;109:1370-84.
43. Russell AJ, DuVall M, Barthel B, et al. Modulating fast skeletal muscle contraction protects skeletal muscle in animal models of Duchenne muscular dystrophy. J Clin Invest. 2023:133.
44. Hamm SE, Yuan C, McQueen LF, et al. Prolonged voluntary wheel running reveals unique adaptations in mdx mice treated with microdystrophin constructs ± the nNOS-binding site. Front Physiol. 2023;14:1166206.
45. García-Castañeda M, Michelucci A, Zhao N, Malik S, Dirksen RT. Postdevelopmental knockout of orai1 improves muscle pathology in a mouse model of Duchenne muscular dystrophy. J Gen Physiol. 2022:154.
46. Lindsay A, Larson AA, Verma M, Ervasti JM, Lowe DA. Isometric resistance training increases strength and alters histopathology of dystrophin-deficient mouse skeletal muscle. J Appl Physiol. 2019;126:363-75.
47. Addinsall AB, Forgan LG, McRae NL, et al. Treatment of dystrophic mdx mice with an ADAMTS-5 specific monoclonal antibody increases the ex vivo strength of isolated fast twitch hindlimb muscles. Biomolecules. 2020;10:416.
48. Ramos JN, Hollinger K, Bengtsson NE, Allen JM, Hauschka SD, Chamberlain JS. Development of novel micro-dystrophins with enhanced functionality. Mol Ther. 2019;27:623-35.
49. Monceau A, Moutachi D, Lemaitre M, et al. Dystrophin restoration after adeno-associated virus U7-mediated dmd exon skipping is modulated by muscular exercise in the severe D2-mdx Duchenne muscular dystrophy murine model. Am J Pathol. 2022;192:1604-18.
50. Hakim CH, Yang HT, Burke MJ, et al. Extensor carpi ulnaris muscle shows unexpected slow-to-fast fiber-type switch in Duchenne muscular dystrophy dogs. Dis Model Mech. 2021:14.
51. Riddell DO, Hildyard JCW, Harron RCM, et al. Longitudinal assessment of skeletal muscle functional mechanics in the DE50-MD dog model of Duchenne muscular dystrophy. Dis Model Mech. 2023;16:dmm050395.
52. Bukovec KE, Hu X, Borkowski M, Jeffery D, Blemker SS, Grange RW. A novel ex vivo protocol to mimic human walking gait: implications for Duchenne muscular dystrophy. J Appl Physiol. 2020;129:779-91.
Cite This Article

How to Cite
Download Citation
Export Citation File:
Type of Import
Tips on Downloading Citation
Citation Manager File Format
Type of Import
Direct Import: When the Direct Import option is selected (the default state), a dialogue box will give you the option to Save or Open the downloaded citation data. Choosing Open will either launch your citation manager or give you a choice of applications with which to use the metadata. The Save option saves the file locally for later use.
Indirect Import: When the Indirect Import option is selected, the metadata is displayed and may be copied and pasted as needed.
About This Article
Special Issue
Copyright
Data & Comments
Data
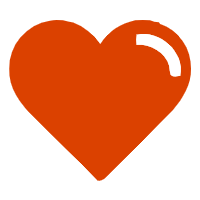
Comments
Comments must be written in English. Spam, offensive content, impersonation, and private information will not be permitted. If any comment is reported and identified as inappropriate content by OAE staff, the comment will be removed without notice. If you have any queries or need any help, please contact us at support@oaepublish.com.