Recent progress of molybdenum-based nitrides/carbides catalysts for lithium-sulfur batteries
Abstract
Lithium-sulfur (Li-S) batteries stand out due to their high theoretical energy density, natural abundance of sulfur, and cost-effectiveness. Despite these advantages, challenges such as the shuttle effect of lithium polysulfides, electrode degradation, and safety concerns hinder their commercialization. Recent advances have focused on integrating molybdenum-based nitrides and carbides to address these issues. These materials offer advantages such as tunable compositions, adjustable lattice structures, semi-metallic conductivity, and enhanced catalytic activity and electron transport. In this mini-review, we delve into the unique physicochemical properties of molybdenum-based nitrides and carbides and the associated composites and their roles in improving the properties of Li-S batteries and discuss their applications as sulfur cathodes and interlayers, mechanisms of lithium polysulfides adsorption, and effects on reaction kinetics. This review aims to consolidate existing knowledge, identify research gaps, and inspire future advancements in Li-S battery technology, paving the way for high-performance, sustainable energy storage solutions.
Keywords
INTRODUCTION
The global market for energy storage systems (ESSs) has undergone rapid expansion over the past few decades. However, current ESSs are struggling to meet the growing demand for grid-connected storage solutions[1-4]. As a result, there is an urgent need for batteries to offer higher energy and power densities in addition to reduced production costs[5]. Among the various battery designs, lithium-sulfur (Li-S) batteries constitute a promising option due to their high theoretical energy density of 2,600 Wh kg-1, which is more than five times that of traditional Li-ion batteries. Additionally, the sulfur cathode provides advantages such as natural abundance, environmental friendliness, and cost-effectiveness[6-8].
The commonly accepted reaction mechanism in Li-S batteries involves the transfer of multiple electrons[9,10], characterized by a series of sulfur-species reactions and the formation of soluble multiple intermediates during charging and discharging cycles [Figure 1A][11]. During lithiation, sulfur (density:
Efforts have been made to address these challenges, such as designing anchoring hosts[16] or modifying separators[17]. Early attempts have focused on integrating sulfur into porous carbon-based materials such as graphene foam[18], porous carbon[19], and carbon nanotube (CNT) networks[20], which provide physical confinement for LiPSs. However, the weak intermolecular interactions between nonpolar, hydrophobic carbon hosts and polar, hydrophobic LiPSs are insufficient to prevent the shuttle effect. Alternatively, polar materials such as transition metal oxides, sulfides, and carbides have been proposed to enhance the adsorption of LiPSs, although improvements have been modest[21-23]. Recently, strategies targeting the acceleration of the conversion between LiPSs and Li2S2/Li2S have been developed[24] by using transition metal compound nanomaterials, including oxides[25,26], sulfides[27-31], selenides[32,33], nitrides[34-36], carbides[37,38], phosphides[39-41], and alloys[42,43]. Other effective approaches include modifying separators to confine LiPSs to the cathode side to mitigate the shuttle effect and protect the Li metal anode. Overall, the key strategy aims at integrating functional materials that provide enhanced conductivity, efficient physical or chemical capture of LiPSs, and/or effective catalytic conversion of LiPSs to improve their properties[44-46].
Among the different types of metallic compounds, Molybdenum (Mo), a silver-gray metal obtained as a by-product of copper/tungsten mining, sees China - a global leader in Mo resources - produce over 100,000 metric tons annually[47]. Its Pt-like electronic structure drives unique physicochemical properties, enabling key applications in electrocatalytic hydrogen production and Li-S batteries. Additionally, Mo-based materials have attracted particular attention due to their tunable compositions, adjustable lattice structures, and variable valence states of Mo centers. These characteristics allow for multiple interactions, including polar adsorption, Lewis acid-base interactions, and conversion reactions, to mitigate the shuttle effect associated with LiPSs[48,49]. Currently, most related research and reviews concentrate on semiconducting Mo-based materials with conventional electronic structures[50]. Nonetheless, The metallic-like electronic structures of MoCx and MoNx deliver high conductivity (e.g., MoCx: ~2,000 S/cm), effectively lowering charge transfer resistance in sulfur cathodes[51]. Nonmetallic elements in their Mo-Mo lattices elongate metal bonds and contract Mo d-orbital electrons, raising d-orbital electron density near the Fermi level and imparting Pt-like catalytic activity[52]. These properties enable MoCx and MoNx to act as both conductive scaffolds and catalytic centers, directly mitigating Li-S battery limitations such as polysulfide shuttling, sluggish kinetics, and high-sulfur-loading instability. Although Mo-based nitrides and carbides possess unique physicochemical properties that make them promising candidates for catalysis and energy storage, they have not been extensively reviewed in the context of Li-S batteries.
In this review, we present the first comprehensive overview of the latest advancements in Mo-based nitrides and carbides for Li-S batteries, with a particular focus on their synergistic effects in electrocatalysis, state-of-the-art doping/interface engineering strategies, and future prospects in solid-state devices, we focus on the latest advancements in Mo-based nitrides and carbides for Li-S batteries. We begin by elucidating the physicochemical properties and catalytic activities of these materials, providing detailed discussions of their properties and activities, and highlighting their mechanisms and properties. Subsequently, we examine the design and application of Mo-based nitrides/carbides in Li-S batteries, including their roles as sulfur cathodes and interlayers for separator membranes. We delve into the interactions between these compounds and LiPSs and investigate the fundamental mechanisms for enhanced adsorption and reaction kinetics. Furthermore, we explore how microenvironments in the composite affect the catalytic ability in the conversion of polysulfides. Finally, we discuss the future development and prospects of advanced Mo-based nitrides and carbides for Li-S batteries. By providing a comprehensive overview of Mo-based nitrides and carbides for Li-S batteries, we aim to consolidate existing knowledge, identify research gaps that must be overcome prior to commercialization, and inspire future innovations in energy storage technologies.
OVERVIEW OF MO-BASED CARBIDES/NITRIDES
Molybdenum carbide MoCx are notable examples of platinum-like, Mo-based compounds, celebrated for their exceptional catalytic activity, which stems from their platinum-like electronic structures. MoCx, as carbides of early transition metals (group IV to VI), are interstitial alloys where carbon atoms are embedded in the lattice interstices of Mo atoms[53]. Their crystal phases [Figure 2A] include α-MoC1-x (0 < x < 0.5),
Figure 2. Crystal structures and electronic structures of two typical molybdenum carbide and nitride. (A) Crystal structure of molybdenum carbide. Reproduced with permission from Ref.[54] Copyright 2014, Wiley-VCH. (B) Electronic band structure of Mo2C calculated with (left) VASP and (right) WIEN2k. Reproduced with permission from Ref.[57] Copyright 2022, American Chemical Society. (C) Crystal structures of molybdenum nitride. Reproduced with permission from Ref.[58] Copyright 2016, Wiley-VCH. (D) Calculated density of states (DOS) of MoN. The orange-shaded areas highlight the DOS contribution near the Fermi level. Reproduced with permission from Ref.[59] Copyright 2014, The Royal Society of Chemistry.
Experimental data and theoretical calculations suggest that the Mo-C bond in MoCx exhibits a significant ionic characteristic, with electrons transferring from Mo to C within a range of zero to two electrons. This electron transfer leads to lattice expansion, d-band contraction, and a larger density of states (DOS) near the Fermi level. These band structure changes enhance the surface and adsorption properties, electron transfer, and reaction kinetics. Such characteristics are crucial to the conversion of LiPSs in batteries by mitigating the shuttle effect, a common challenge to Li-S batteries impacting capacity and efficiency. In the meantime, after carbon insertion, the shortest Mo-Mo bond distance expands from 272 to 300 pm, resulting in d-band contraction in metallic Mo and boosting the DOS of Mo atoms near the Fermi level. Comparison of band structure calculations for Mo (110) and Mo-terminated β-Mo2C (100) demonstrates that Mo-C bonding broadens the d-band and redistributes the DOS below (< -4 eV) and above (> 3.5 eV) the Fermi level[56]. This redistribution, with the d-band center moving closer to the Fermi level, imparts MoCx with an electronic structure similar to noble metals[57] [Figure 2B]. Consequently, the unique electronic structure and catalytic activity of molybdenum carbide make it highly effective in addressing key issues of Li-S batteries, such as improving reaction kinetics and minimizing the shuttle effect, in order to enhance the battery capacity and cycling life for S cathodes.
Similarly, MoNx, structurally related to molybdenum carbides, represents a class of interstitial compounds with solid-state chemical properties akin to the parent metal. Formed by small-radius nitrogen (N) atoms in the Mo atomic lattice, MoNx, as depicted in [Figure 2C], involves Mo atoms adopting face-centered cubic (fcc) and hexagonal close-packed (hcp) structures[58], with N atoms occupying octahedral interstitial sites within both fcc and hcp lattices. The insertion of N atoms introduces tensile strain to the Mo atomic lattice, thus reducing the overlap of adjacent metal atoms' d-bands and increasing the DOS near the Fermi energy level[59]. This alteration produces an electronic structure that resembles platinum (Pt) [Figure 2D]. The primary types of bonding in Mo nitride include metallic bonds between Mo atoms, covalent bonds between Mo and N atoms, and ionic bonds formed through charge transfer between Mo and N atoms. Owing to these unique structural properties, MoNx exhibits metallic conductivity, excellent chemical stability, and high catalytic activity, making it widely applicable to Li-S batteries.
MOLYBDENUM CARBIDE CATALYSTS IN LI-S BATTERIES
Mo2C catalysts
As discussed above, Mo2C exhibits excellent metallic conductivity and remarkable catalytic activity due to its platinum-like electronic structure. Recently, it has gained attention as a promising polar catalyst for both catalytic hosts in the cathode and the modified layers on the separators in Li-S batteries. As for the former, Sun et al. Have synthesized Mo2C@C as a cathode sulfur host in Li-S batteries[60]. Mo2C undergoes sulfidation upon contact with sulfur to produce a sulfided surface (S-Mo2C) with moderate adsorption energy (2-3 eV) and suppressed shuttle effect. Additionally, density-functional theory (DFT) calculations show that the oxidation energy barrier of Li2S on the sulfided Mo2C surface is only 0.38 eV, which is significantly lower than that of traditional carbon-based materials. The reduction in the energy barrier accelerates the redox reactions of LiPSs. At a rate of 0.2 C, the initial specific capacity reaches
However, despite its high catalytic activity, the relatively small specific surface area of Mo2C limits the exposure of active sites, resulting in reduced adsorption and catalytic efficiency. Therefore, controlling the particle size of molybdenum carbide while improving its conductivity and dispersibility is theoretically advantageous by exposing more active sites to maximize the electrocatalytic activity in Li-S batteries. For instance, Razaq et al. have prepared a composite with Mo2C nanoparticles anchored on CNTs (CNT/Mo2C) as a sulfur host by annealing CNTs and ammonium molybdate at 800 °C [Figure 3A][61]. Mo2C nanoparticles are anchored on CNTs, provide polar sites for LiPSs adsorption and facilitate their conversion
Figure 3. Enhancing the properties of LSBs by the host design and separator modification. (A) Schematic illustration of the synthesis process of Mo2C/CNT and S-Mo2C/CNT. (B) Illustration of the promoted adsorption and the redox reaction of polysulfides on
Further enhancements in the electrochemical activity of Mo2C nanomaterials in Li-S batteries have been achieved by ion doping, which induces atomic redistribution and exposes abundant edge sites. For example, Wang et al. have synthesized P-doped Mo2C nanoparticles anchored on carbon nanofibers (CNFs) by an organic-inorganic nanohybridization process[63]. By modulating the electronic structure of Mo2C through phosphorus doping, the electron density increases, and P-Mo2C possesses stronger LiPSs adsorption and enhanced sulfur redox kinetics. DFT calculations reveal that there are abundant active sites on the P-doped Mo2C surfaces, thereby optimizing both the formation and decomposition processes of Li2S. These findings illustrate that P-doped Mo2C offers enhanced LiPSs adsorption capabilities and improved sulfur redox kinetics [Figure 3E]. Chen et al. engineered B-doped metastable cubic Mo2C (δ-B-Mo2C/NCNT) as a sulfur host[64]. Its 2D nanosheet-assembled tubular structure enables rapid LiPS electron transfer and confinement. DFT calculations reveal B-doping-induced phase transition shifts the Mo d-band center upward, enhancing Mo(d)-S(p) orbital hybridization to accelerate kinetics. The δ-B-Mo2C/NCNT achieves 606.3 mAh g-1 at
Similarly, Li et al. designed cobalt-doped β-Mo2C spheres (Co-Mo2C@C) as sulfur nano-reactors to optimize the growth of Li2S1/2 and to accelerate the conversion kinetics from Li2S2 to Li2S[65]. DFT calculations indicate that Co-Mo2C@C exhibits strong interactions with Li2S2, shortening the Li-S bond length, which makes it more prone to breaking and ultimately forming Li2S. Furthermore, Co-Mo2C@C lowers the energy barrier for the Li2S2-to-Li2S conversion and enhances both electron transfer and ion diffusion rates. As a result, the Co-Mo2C@C/S cathode delivers an excellent specific capacity of 1,200 mAh g-1 at 0.1 C, and it also demonstrates outstanding cycling stability.
These examples highlight three doping approaches: anionic (P) and cationic (B). Both strategies modify electronic structures, induce lattice strain, and optimize active sites for synergistic enhancement. Transition metal compounds, leveraging their d-orbital tunability, enable precise band structure adjustments via doping, significantly boosting sulfur redox kinetics.
Building on the effectiveness of Mo2C as host materials in enhancing Li-S batteries, attention has been paid to the critical role of the modification of separators[66,67]. Positioned between the sulfur cathode and the lithium anode, the separator acts as the sole pathway for the diffusion of soluble LiPSs from the cathode to the anode, earning it the moniker "auxiliary electrode". Recent advancements in separator design enable the suppression of the shuttle effect while improving the electrochemical kinetics of Li-S batteries. Yu et al. have performed DFT calculations to predict that Mo2C can accelerate Li+ diffusion to achieve uniform lithium deposition[68]. Experimental studies have demonstrated the successful anchoring of P-doped Mo2C quantum dots (MQDs) on nitrogen-doped graphene (MQD@NG). These polar MQDs exhibit strong chemical adsorption toward LiPSs and provide rapid diffusion channels for Li+ transport [Figure 3F and G], consequently facilitating uniform Li+ flow across the electrode surface and dendrite-free, uniform lithium deposition.
By applying these advancements to Mo2C, Li-S batteries deliver enhanced performance, thus addressing key challenges such as the shuttle effect and uniform lithium deposition. This holistic approach underscores the potential of Mo2C and innovative separator designs to revolutionize Li-S batteries.
MoC catalysts
Both hexagonal η-MoC and fcc α-MoC1-x exhibit an ABCABC stacking sequence. Although they have lower electronic conductivity and surface stability compared to the ABAB-stacked β-Mo2C with metallic planes, the ABCABC stacking introduces more diverse atomic layer positions, resulting in a greater variety of active sites on the surface. These diverse active sites are effective in adsorbing and activating LiPSs to enhance the catalytic conversion efficiency. Moreover, the complex stacking sequence facilitates the formation of surface defects and edge sites, which typically exhibit higher chemical activity compared with Mo2C, contributing to improved adsorption and conversion capabilities for LiPSs. The research associated with the MoC has mainly focused on the design of the nanostructured composite hosts to enhance the exposed catalytic sites. Shi et al. have embedded necklace-like MoC nanoparticles into an interconnected CNF network[69]. The uniformly distributed MoC nanoparticles serve as chemical traps and electrocatalysts to adsorb LiPSs and accelerate the redox reactions. In addition, the interconnected structure facilitates the rapid transport of Li+ and electrons throughout the electrode, which is particularly beneficial for cathodes with high sulfur loading
Figure 4. MoCx-based electrocatalyst used for LSBs. (A) Schematic diagram of the preparation of the MoC@N-CNF freestanding electrode. (B) Role of necklace-like MoC in LiPS immobilization and transformation. Reproduced with permission from Ref.[69] Copyright 2019, The Royal Society of Chemistry. (C) Static adsorption experiment of MoC1−x@NCF and NCF in Li2S6 solution and corresponding UV-vis absorption spectrum. (D) Cyclic characteristics of MoC1-x@NCF//PP cell at 0.1 C with a high sulfur loading and lean electrolyte. Reproduced with permission from Ref.[71] Copyright 2023, Wiley-VCH.
Downsizing molybdenum carbide (MoC) to enhance its specific surface area through microstructure and nanostructure design is inherently constrained. While higher MoC loadings allow for the rapid conversion of adsorbed LiPSs, the effectiveness of this process is significantly hampered by the smaller number of active sites and surface area. This limitation ultimately compromises the catalytic efficiency of the MoC. Therefore, it is crucial to determine the optimal MoC loading on the cathode to optimize the catalytic activity. Ji et al. have fabricated CNFs@MoC composites with different MoC loadings using electrospinning combined with carbothermal reduction[70]. By adjusting the calcination temperature and molybdenum acetylacetonate content, the adsorption of LiPSs is improved with increasing MoC loading. Among the various composites, CNFs@MoC-37% has the highest Li2S deposition capacity
Similar to Mo2C, MoC is also used to modify the separator as a barrier to alleviate the shuttle effect. For instance, Li et al. have synthesized highly dispersed α-phase molybdenum carbide nanocrystals embedded in a porous nitrogen-doped carbon framework (α-MoC1-x@NCF) using the MOFs as templates[71]. These composites are employed as multifunctional interlayers for separators in Li-S batteries. Benefiting from the synergistic capture and catalytic effects of the MoC1-x@NCF interlayer on LiPSs [Figure 4C], even at high sulfur loading (6.0 mg cm-2) and lean electrolyte conditions (E/S ≈ 5.8 μL mg-1) [Figure 4D], the assembled Li-S batteries show an exceptionally high capacity of 5.2 mAh cm-2.
Mo2C/MoC heterostructure catalysts
Mo2C and MoC have distinct advantages and limitations in Li-S batteries, and their integration can yield superior performance. Mo2C, characterized by its ABAB stacking pattern, boasts high metallic conductivity, excellent surface stability, rapid electron transport, and efficient polysulfide conversion. However, the limited range of active sites may constrain its effectiveness in adsorbing and catalyzing LiPSs. Conversely, MoC with the ABCABC stacking offers a diverse array of active sites and promotes the formation of surface defects to enhance LiPSs adsorption and catalytic conversion. Despite these benefits, the lower electronic conductivity and surface stability of MoC compared to Mo2C can degrade the electrochemical properties. The Mo2C/MoC heterostructure capitalizes on the strength of both materials[72] to deliver enhanced conductivity, abundant active sites, and better LiPSs management to address the shuttle effect, improve sulfur utilization, and enable stable long-term cycling. The synergistic effects at the interface further boost the catalytic performance and structural stability, rendering Mo2C/MoC heterostructures a promising solution for high-performance Li-S batteries.
For instance, Han et al. have designed a mixed-valence nanoscale Mo2C-MoC/C cathode for Li-S batteries, where the mixed valence (Mo2+ and Mo3+) within the Mo2C-MoC structure significantly enhances the electrical conductivity and reaction kinetics to counteract the insulating nature of sulfur and lithium sulfide[73]. This structure shows stronger adsorption and catalytic conversion activity for polysulfides than single-valence Mo2C, yielding an initial discharge capacity of 870.4 mAh·g-1 at 0.2 C, compared to
MOLYBDENUM NITRIDE CATALYSTS IN LI-S BATTERIES
Mo2N catalysts
Spurred by the advancements in Molybdenum carbides for Li-S batteries, molybdenum nitride (Mo2N) emerges as another candidate offering exceptional catalytic activity and chemical stability rivaling noble metals. The remarkable properties of Mo2N stem from its unique electronic structure shaped by Mo-N bonds in the crystal lattice. During the formation of the Mo-N bond, nitrogen atoms occupy interstitial sites of the molybdenum lattice and expand the interatomic distance between adjacent Mo atoms. The restructuring significantly enhances the DOS near the Fermi level, leading to active electron transfer properties and positioning Mo2N as an excellent catalyst material. For instance, Jiang et al. have synthesized mesoporous Mo2N with an average pore size of 8.6 nm using silica as a template with strong adsorption capabilities for LiPSs [Figure 5A and B][75]. This mesoporous structure has superior electrochemical characteristics such as high-capacity retention of 92.0% (914 mAh·g-1) after 100 cycles at 0.5 C, which outperforms non-porous Mo2N cathodes.
Figure 5. Mo2N used as an effective sulfur host or interlayer for LSBs. (A) Charging/discharging process of the mesoporous Mo2N/S cathode. (B) TEM image of mesoporous Mo2N. Reproduced with permission from Ref.[75] Copyright 2018, Elsevier. (C) Schematic diagrams of the synthesis of Mo2N@NG. (D) High-resolution TEM image of Mo2N@NG. (E) Schematic illustration of the preparation stages for MoS2 QDs-NRGO. (F) Cyclic voltammetry curves of the Li2S6 symmetric cell. Reproduced with permission from Ref.[76] Copyright 2022, Wiley-VCH. (G) PDOS of QMo2N and QMo2N-V. (H-J) In situ time-resolved Raman contour mappings of the cells charged and discharged at 0.5 C with QMo2N-V modified separators. H Discharging and H charging J Cycling properties of QMo2N-V. Reproduced with permission from Ref.[77] Copyright 2022, Elsevier.
To further enhance the catalytic properties of Mo2N, quantum dots (QDs) have been introduced to take advantage of the abundant active sites and redox catalytic activity. Ma et al. have constructed Mo2N QDs anchored on N-doped graphene oxide (Mo2N@NG) by simple reflux-calcination [Figure 5C and D] to serve as a multifunctional interlayer on the polypropylene (PP) separator[76]. The well-dispersed Mo2N QDs in the NG framework exhibit strong chemical adsorption and affinity toward Li+ [Figure 5E], as well as excellent electrocatalytic activity for LiPSs conversion. Cyclic voltammetry reveals that the Mo2N@NG electrode outperforms the NG electrode with a larger peak area and higher current response, confirming its ability to accelerate the anchoring-diffusion-transformation process of LiPSs [Figure 5F]. This design suppresses the shuttle effect and enhances the redox kinetics, leading to improved sulfur utilization and stable cycling characteristics.
However, despite these advancements, optimizing the intrinsic catalytic activity of Mo2N remains critical for achieving high-energy-density Li-S batteries with reduced electrocatalyst loadings. Engineering atomic vacancies is a powerful strategy to modulate the electronic structure of Mo2N and enhance both the chemisorption and catalytic conversion of LiPSs. Yang et al. have synthesized nitrogen vacancy-rich Mo2N QDs (QMo2N-V) by spray drying[77]. These QDs are integrated into mesoporous carbon microspheres and act as efficient microreactors to capture diffusing LiPSs and facilitate sulfur reutilization. DFT calculations reveal that nitrogen vacancies shift the d-band center of Mo2N from -1.90 to -1.66 eV [Figure 5G] to improve LiPSs adsorption and reaction kinetics [Figure 5H and I]. Li-S batteries with QMo2N-V-modified separators show a reversible capacity of 510 mAh·g-1 for 400 cycles at 4.0 C and an impressive areal capacity of 6.6 mAh·cm-2 for a high sulfur loading (8.1 mg·cm-2) and lean electrolyte conditions [Figure 5J]. Similarly, Cheng et al. have synthesized oxygen-modified Mo2N nanoclusters (C-Mo2N-O) by metal-organic coordination polymerization to uniformly load the clusters onto the mesoporous carbon sphere frameworks[78]. Guided by the Sabatier principle, the surface oxygen atoms weaken the interactions between sulfur species and Mo atoms to balance the adsorption strength and avoid site poisoning. This moderated interaction promotes efficient reversible catalysis. All in all, advancements of Mo2N by means of structural engineering (e.g., mesoporous frameworks, QDs, and atomic vacancies) highlight its transformative potential in addressing the challenges of polysulfide management in the pursuit to achieve superior capacity retention and the practical deployment of high-sulfur-loading Li-S batteries.
MoN catalysts
The molybdenum nitride phase, MoN, has garnered attention for its distinct advantages. While Mo2N has remarkable chemical stability, strong LiPSs adsorption, and enhanced catalytic activity, MoN, with its hexagonal close-packed (HCP) structure, brings additional benefits such as its highly ordered atomic arrangement, superior electrical conductivity, and elevated Mo oxidation state. These properties render MoN a promising candidate to further improve the electron transport and catalytic efficiency in Li-S batteries. Liu et al. have embedded MoN QDs into a dual-channel ordered mesoporous carbon composite (MoN@CMK-5)[79]. The carefully designed bimodal pore structure of MoN@CMK-5 offers a large specific surface area and enhanced electrolyte mass transport, while the high intrinsic catalytic activity facilitates the rapid conversion of polysulfides. This "adsorption-conversion-mass transport" synergy gives rise to outstanding electrochemical characteristics, including an initial capacity of 1,582 mAh·g-1 at 0.1 C and a capacity decay rate of just 0.027% per cycle after 1,000 cycles at 5 C [Figure 6A]. Furthermore, theoretical calculations reveal that MoN@CMK-5 has a low activation energy for Li2S decomposition
Figure 6. MoN used as an effective sulfur host or interlayer for LSBs. (A) Cyclability of MoN-X@CMK-5 at 5 C. (B) Binding energy for different polysulfides for MoN materials. (C) Reaction energy barrier of Li2S → LiS-+ Li+ harvested from carbon and MoN substrates. Reproduced with permission from Ref.[79] Copyright 2022, Elsevier. (D) Schematic illustration of the polysulfide anchoring and enhanced conversion on the surface of exposed MoN nanosheets. (E) Oxidation peak I (Li2S → Li2Sx) and reduction peak IV
In comparison with QDs, 2D materials excel in creating continuous conductive networks, suppressing polysulfides through large and stable interfaces, and providing better structural stability and scalability. They are particularly suitable due to their long-term cycling stability and large-scale electrode fabrication. Tan et al. have synthesized molybdenum nitride nanosheets (MoN@CNFs) on CNFs [Figure 6D][80]. The unique nanosheet morphology provides abundant active sites, and the interconnected CNF network ensures efficient electron transport. MoN@CNFs exhibit the smallest Tafel slopes during oxidation and reduction
To further optimize bifunctional catalysis for high-performance Li-S batteries, Li et al. have fabricated a layered MoN-C-MoN structure to leverage the strong adsorption capability and high catalytic activity while enhancing electron transport through the conductive carbon sheets[81]. This layered design maximizes surface exposure for adsorption and catalysis and promotes efficient bidirectional conversion between S and Li2S with minimal reaction overpotentials [Figure 7A and B].
Figure 7. Application of molybdenum nitride with bidirectional catalytic functionality in LSBs. (A) Schematic illustration of bifunctional catalysis for MoN-C-MoN. (B) CV curves of symmetrical cells. Reproduced with permission from Ref.[81] Copyright 2020, Wiley-VCH. (C) Schematic Illustration of bifunctional catalysis for MCN-Mo and binding energy and electron cloud density after MoN Adsorption. (D) Fermi Level of MoN. Reproduced with permission from Ref.[82] Copyright 2022, The Royal Society of Chemistry.
Liu et al. prepared MoXn (X = O, S, N, and P) compounds featuring fixed Mo cations and various anions, and investigated the influence of these anions’ p-bands on Li-S electrochemistry[82]. DFT calculations reveal that shifting the p-band center leads to different interfacial electron-transfer dynamics, resulting in diverse catalytic activities for polysulfide conversion. The results show that Li-S batteries with a MoN-modified separator exhibit relatively better electrochemical performance, attributed to the narrower energy gap between the metal 3d center and the anion 2p center in MoN compared to the other compounds. Both experimental and computational data confirm that this narrow band gap effectively lowers the decomposition barrier of Li2S. In particular, the significantly improved kinetics further enhances overall performance [Figure 7C and D].
In addition to the intrinsic advantages, the catalytic activity of MoN can be further improved by metal heteroatom doping, which modulates its electronic structure and enhances LiPSs adsorption and conversion. Kong et al. have synthesized Co/Mo2N composites using a hydrothermal and ammonia nitridation approach[83]. The combination of Co and MoN provides synergistic effects to improve the Li2S nucleation kinetics and sulfur redox reactions. [Figure 8A confirms Co → MoN electron transfer (yellow/blue regions: accumulation/depletion), creating a built-in field that streamlines electron transport for LiPSs redox. MoN shows superior Li2S adsorption (-3.28 eV), preventing intermediate loss. [Figure 8B reveals MoN lowers Li2S2 → Li2S conversion energy barriers (ΔG), a rate-limiting step. Reduced barriers accelerate kinetics, enhancing battery performance (capacity, rate capability). As a result, the Li//Li symmetrical cell with Co/Mo2N-coated separators shows stable plating and stripping for 800 h.
Figure 8. DFT of Co/Mo2N (A) Charge density difference between Co and MoN (yellow/blue regions indicate electron accumulation/depletion) and optimized Li2S configurations and binding energies on Co, MoN, and Co/MoN. (B) Gibbs free energy of LiPSs conversion on Co (111), MoN (200), and Co/MoN; insets: LiPSs adsorption geometries on each surface. Reproduced with permission from Ref.[83] Copyright 2023, Wiley-VCH.
In summary, MoN builds upon the successes and limitations of Mo2N by offering superior conductivity and catalytic activity, making it a powerful candidate for next-generation Li-S batteries. Through innovative structural designs, such as mesoporous composites and carbon-based hybrids, along with electronic modulation strategies such as heteroatom doping, MoN has significant potential to address the challenges posed by polysulfide management, sulfur utilization, and long-term cycling stability.
Mo2N/MoN heterostructures catalysts
Mo2N and MoN are both promising materials for Li-S batteries due to their excellent catalytic properties and high electrical conductivity, which enhance the conversion kinetics of LiPSs and improve overall battery performance. Mo2N is particularly noted for its strong polysulfide adsorption capabilities[84] which help mitigate the shuttle effect, while MoN provides robust structural stability and mechanical strength[85]. However, individually, these materials face challenges such as limited surface area and potential stability issues under prolonged cycling conditions. In the process of preparing MoNx through nitridation, insufficient nitridation often results in a mixed phase of Mo2N and MoN, leading to the formation of abundant grain boundaries between them. These grain boundaries play a crucial role in modulating the surface electronic structure and enhancing catalytic adsorption capabilities[86,87]. By building Mo2N/MoN heterostructures, we can leverage the complementary strengths of both materials - combining their catalytic efficiencies, electrical conductivity, and adsorption properties - while addressing their individual limitations. This synergistic approach can result in enhanced reaction kinetics, improved structural integrity, and, ultimately, more efficient and durable Li-S batteries.
For instance, Yang et al. synthesized hollow microspheres composed of an MoN-Mo2N heterostructure rich in grain boundaries[88]. These grain boundaries serve as 2D nucleation sites, exhibiting strong and rapid adsorption abilities toward LiPSs and guiding the deposition of Li2S around them, thereby effectively preventing catalyst surface passivation. Consequently, these hollow microspheres enable high-capacity Li2S deposition and significantly enhance the conversion kinetics of LiPSs. In assembled batteries featuring an interlayer of these microspheres and CNTs, they demonstrated a low capacity decay of 0.049% per cycle at
MOCX/MONX-CONDUCTIVE COMPOUND COMPOSITE CATALYSTS
Although nanosized MoCx and MoNx exhibit remarkable catalytic abilities for converting polysulfides, they tend to aggregate into larger particles with decreased electrochemical activity. Hence, MoCx and MoNx are typically integrated into a conductive framework, such as carbon materials and metallic compounds, to enhance stability. The catalytic activity of MoCx and MoNx composites is influenced by the chemical and coordination environments. The chemical environment, which includes different phases or dopants, can modify the electronic properties to improve the interactions between the catalyst and reactants. The coordination environment, defined by the arrangement of atoms around active sites, impacts their accessibility and reactivity and promotes more efficient catalytic reactions. Furthermore, synergistic interactions in MoCx and MoNx composites can lead to the development of new active sites, combining the electrical conductivity of MoCx with the adsorption capabilities of MoNx. By thoroughly investigating the microenvironments in the composite, it is possible to improve the catalytic ability and efficiency in applications such as energy storage and conversion systems.
MoCx/MoNX-carbon composites
Combining carbon materials with MoCx and MoNx to fabricate MoCx/MoNx-carbon composites offers significant benefits, particularly for Li-S batteries. Carbon materials possess a high intrinsic surface area, which provides more active sites for catalytic reactions and facilitates the interactions between the catalyst and reactants to improve the efficiency of energy conversion and storage. However, designing micro and nanostructures to increase the surface area of MoCx/MoNx is still challenging. As the sulfur concentration or areal loading in the cathode increases, the availability of sulfur hosting sites becomes insufficient and furthermore, the persistent LiPSs shuttling effect during long-term cycling severely compromises the battery lifetime.
Owing to the large specific surface area and excellent electrical conductivity of carbon materials, integrating MoCx/MoNx with carbon presents an effective strategy to improve the properties of Li-S batteries. Three-dimensional (3D) carbon architectures with optimized pore structures are the ideal substrates as they allow large sulfur loading and accommodate the volumetric expansion during cycling. For instance, Niu et al. have designed a freestanding Mo2C/graphene/N-doped carbon foam composite (GCF-G@Mo2C) featuring an agar-like porous structure[89]. Here, graphene sheets wrapped around N-doped carbon bubbles enable rapid electron and ion transport. Experiments and first-principles calculations reveal that the Mo2C nanoparticles, acting as chemical anchoring centers, exhibit a high affinity for polysulfides and accelerate the redox conversion through catalytic effects. Consequently, the GCF-G@Mo2C battery shows an initial capacity of 862 mAh g-1 and maintains a capacity of 597 mAh g-1 after 600 cycles at 1 C, with a decay rate of only 0.051%, demonstrating excellent cycling stability.
The porous structure of carbon enhances its catalytic ability by increasing the surface area, improving mass transport, and ensuring efficient dispersion and accessibility of active sites. MOFs, with their well-defined porous structures and crystallinity, offer an alternative approach to constructing 3D porous carbon architectures. Wang et al. have synthesized MoN@N-doped carbon (MoNNC) porous octahedra using a MOF precursor by high-temperature thermal annealing, etching, and nitridation [Figure 9A][90]. Cyclic voltammetry conducted on the symmetrical cell in the Li2S6-containing electrolyte demonstrates that MoNNC promotes the chemisorption and conversion of LiPSs. The MoNNC/S cathode, with a 77.0 wt% sulfur loading, shows a capacity retention of 88.0% at 0.5 C and maintains a reversible capacity of
Figure 9. Application of MoCx/MoNx composites with high-specific-surface-area carbon materials in Li-S batteries. (A) Synthesis process for MoN-NC. Reproduced with permission from Ref.[90] Copyright 2019, The Royal Society of Chemistry. (B) Schematic illustration of fabrication strategy of Mo2C@BOC host. (C) Precipitation profiles of Li2S. Reproduced with permission from Ref.[92] Copyright 2023, Elsevier.
In practical applications of Li-S batteries, the economic significance of large-scale, controllable synthesis of heteroatom-doped carbon materials must be considered. Using a biological carbon source, Wang et al. have prepared ultrafine Mo2C nanocrystals anchored on a bio-oil-derived 3D layered carbon matrix
MoCX/MoNX-metallic compound composites
In addition to carbon materials, integrating metallic compounds with MoCx and MoNx in Li-S battery composites can address several key challenges in battery technology. The integration enhances the catalytic activity, electrical conductivity, and structural stability, leading to more efficient and durable energy storage. Typically, single materials have limited adsorption and catalytic capabilities for LiPSs. However, by constructing heterostructures composed of two different metal compounds, the chemical confinement and conversion of polysulfides are synergistically enhanced[93,94]. These materials with heterostructures possessing superior electrical properties can be directly used as electrode materials or additives to boost the battery performance. For instance, in Li-S batteries, heterostructures help anchor LiPSs and catalyze the accelerated conversion through the built-in electric fields to achieve the synergistic "adsorption-catalysis" effect. Unlike alloying, which combines multiple metals, heterostructure engineering involves hybridizing different types of compounds with tightly bonded interfaces[95].
As discussed previously, MoCx/MoNx exhibits high conductivity and catalyzes the rapid conversion of LiPSs in Li-S batteries. In heterostructures based on highly conductive transition metal compounds, electrons transfer swiftly along the heterointerface. The distinct components of the heterojunction create an intrinsic electric field that facilitates rapid Li+ diffusion, resulting in superior catalytic performance. For instance, Zhao et al. have developed Mo2N-MoC/NC nanomaterials with dense heterointerfaces[96]. The Mo2N-MoC interface generates a strong built-in electric field to enhance electron migration and the redox reactions of LiPSs [Figure 10A and B]. These properties make Mo2N-MoC/NC nanobelts ideal for separator modification, effectively capturing, diffusing, and converting soluble LiPSs into solid
Figure 10. MoCx/MoNx heterostructures for LSBs. (A) Schematic illustration for the efficient trapping-diffusion-conversion of soluble LiPSs to solid Li2S on the Mo2N-MoC/NC-PP. (B) Photograph of the Li2S6 adsorption tests and High-resolution XPS spectra before and after Li2S6 adsorption tests. (C) Long-term cycling performance of the battery with Mo2N-MoC/NC-PP at 1 C. Reproduced with permission from Ref.[96] Copyright 2023, Wiley-VCH. (D) Energy bands between Mo2C and MoS2 before and after the Schottky contact. (E) TDOS for Mo2C-MoS2, MXene Mo2C and MoS2 models. (F) Charge density difference for Mo2C-MoS2 in-plane multi-heterostructures. (Insets) The ball-and-stick model of Mo2C-MoS2 (left) and the illustration of the built-in electric fields (right). Reproduced with permission from Ref.[100] Copyright 2024, Wiley-VCH.
Metallic compounds forming Mott-Schottky heterojunctions with specific compounds enable spontaneous charge migration and separation at the interface, leading to electron enrichment on one side and generation of abundant holes on the other side to create an intrinsic electric field that enhances the redox conversion of LiPSs by optimizing the interfacial electronic structure[98,99]. For example, Li et al. have synthesized Mo2C-MoS2 in-plane multi-heterostructures by the topological conversion of sandwich-like mesoporous Mo2C-SiO2 layers in sulfur vapor, followed by the removal of SiO2[100]. During the conversion, the exposed Mo2C is transformed into the 2H phase MoS2, while the covered Mo2C remains stable, resulting in a layer that combines metallic Mo2C MXene and semiconducting MoS2 [Figure 10D]. The Mo2C-MoS2 layer with multiple heterointerfaces, built-in electric fields, and abundant defects improves the electrochemically active surface area (16.4 mF cm-2) [Figure 10E and F]. This structure not only facilitates the bidirectional sulfur electrochemistry between solid Li2S and soluble LiPSs but also enhances the transfer kinetics of electrons and ions, resulting in a high rate of 642 mAh g-1 at 5 C and long-term cycle lifetime (1,000 cycles at 5 C) in Li-S batteries.
Du et al. proposed a novel heterogeneous catalysis/deposition mechanism to enhance polysulfide conversion[101]. A heterostructured Mo2C/α-MoO3/G nanomaterial was synthesized, featuring crystalline and amorphous phases with electronic delocalization and a built-in electric field. These features boost polysulfide conversion kinetics by increasing reaction rates and lowering activation energies. They also optimize Li2S deposition, yielding a uniform 3D progressive nucleation (3DP) morphology and rapid Li+ diffusion on the catalyst surface. Consequently, even under a lean electrolyte level of 9.3 μL mg-1, the LSB with Mo2C/α-MoO3/G delivers an initial specific capacity of 1,055 mAh g-1 and an areal capacity of
Heterostructures integrate distinct domains with differing properties, achieving synergistic effects through interfacial interactions that exceed simple component mixtures. Their catalytic mechanisms include (1) adsorption-catalysis synergy; (2) tandem catalysis; (3) bidirectional reaction promotion; and (4) electronic structure modulation[102]. Therefore, heterostructures composed of TMCs with different Fermi levels can induce interfacial built-in electric fields and electron transfer, triggering electron redistribution and localized atomic rearrangement. This modulates electronic structures (e.g., band structure, d/p-band centers, electron occupancy, and valence states), significantly enhancing adsorption catalysis, thus suppressing polysulfide shuttling and improving rate/cycling performance[103].
CONCLUSION AND PROSPECTS
In summary, Li-S batteries have emerged as promising candidates for next-generation ESSs because of advantages such as high theoretical energy density, cost-effectiveness, and environmental compatibility. However, their practical application is hindered by intrinsic challenges, including the shuttle effect of LiPSs (LiPSs), sluggish redox kinetics, and the instability of the lithium metal anode. To overcome these obstacles, Mo-based carbides (MoCx) and nitrides (MoNx) have demonstrated exceptional potential as electrocatalysts and structural components due to their unique electronic structure, superior catalytic activity, and high electrical conductivity. The integration of MoCx and MoNx into Li-S battery systems, as cathode hosts, separator modifications, or multifunctional interlayers, has shown significant promise in mitigating the shuttle effect, accelerating LiPSs conversion, and improving sulfur utilization.
Notably, nanostructured Mo-based materials such as Mo2C and MoN and their heterostructures exhibit tunable electronic properties, strong adsorption of LiPSs, and excellent catalytic activity. These materials facilitate rapid polysulfide conversion and uniform lithium deposition, resulting in improved cycling stability and rate performance. Heterostructure engineering, such as combining MoCx and MoNx or integrating them with carbon frameworks and metallic compounds, increases active site exposure, creates built-in electric fields, and optimizes electron/ion transfer kinetics. These advancements have spurred significant breakthroughs, including high capacities, low-capacity fade rates, and excellent long-term cycling stability under high sulfur loadings and lean electrolyte conditions.
Table 1 summarizes the applications of Mo-based compounds with different nonmetallic elements in Li-S batteries. Mo-based materials exhibit diverse chemical compositions and morphological structures, and variations in nonmetallic elements significantly influence their adsorption capacity and catalytic activity toward LiPSs. The modulation of anions alters the Δd-p (representing the p-d hybridization state between Mo and nonmetallic elements), thereby regulating the LiPSs conversion pathways and enhancing reaction kinetics[112-114]. Consequently, Δd-p serves as a more accurate descriptor than the d-band center for evaluating the electrocatalytic performance of materials, as it fundamentally governs the catalytic mechanism. When the active Mo sites in Mo-based compounds interact with Li2S4, electrons transfer from Li2S4 molecules to the catalyst. The Δd-p of the catalyst determines the redistribution of electrons within the compound after thermodynamic adsorption, which in turn dictates catalytic performance. Specifically, catalysts with smaller Δd-p exhibit lower energy barriers for redistributing additional electrons in the system, leading to stronger interactions with Li2S4. This is because the magnitude of Δd-p governs electron transfer during Li2S4 conversion: 1. Catalysts with smaller Δd-p facilitate electron transfer to nonmetallic elements in the compound, increasing the total electron transfer during interactions with Li2S4; and 2. This process lowers the energy level of the antibonding orbitals in S-S bonds, thereby reducing the activation energy for electrochemical catalysis and improving reaction kinetics.
The applications of transition metal compound materials in Li-S batteries
Classification | Material | Structure characteristics | Sulfur loading (mg/cm-2)/(%) | Cycle number (rate) | Initial/final capacity (mAh/g) | Refs. |
Molybdenum carbides | Mo2C/C | Nanoparticles | 1.5 /N/A | 400 (1 C) | 798/612 | [60] |
Mo2C/NG | Quantum dots | 1.5 /N/A | 100 (0.2 C) | 1,230/1,146 | [68] | |
α-MoC1−x/NC | Nanocrystallites | 4.3 | 85 (0.1 C) | 845/785 | [71] | |
Molybdenum nitrides | Mo2N/C | Porous | N/A/N/A | 100 (0.5 C) | 995/914 | [75] |
Mo2N/NG/PP | Quantum dots | N/A | 300 (0.5 C) | 858/706 | [76] | |
MoN/CMK-5 | Bimodal pore structure | N/A/N/A | 1,000 (5 C) | 475/344 | [79] | |
Co/MoN | Nanoparticle | 1.2/N/A | 500 (1 C) | 860/554 | [83] | |
Other molybdenum-based compounds | MoS2/CNT/S | Cross-stacked membrane | 2.6/N/A | 50 (0.2 C) | 855/495 | [104] |
MoO3/CNF | Nanofiber membrane | N/A,/N/A | 500 (0.2 C) | 776/427 | [105] | |
CMoP/CMK/3 | Clusters confined into mesoporous carbon | N/A/70% | 500 (2 C) | 902/811 | [106] | |
CoO/MoO3@NC | Hollow structure | 1.2/ N/A | 100 (0.1 C) | 1,256.8/802.8 | [107] | |
Mo1-xNbxS2HNTs | Nanosheets assembled hollow nanotubes | 3.13/N/A | 47 (0.5 C) | 613/434 | [108] | |
Other transition metal compounds | Fe3O4@GCF | Nanoparticle | 1.5/N/A | 100 (1 C) | 1,245/700 | [109] |
Fe2O3/N-MC | Nanocrystalline structure | 5.1/72.5 | 500 (1 C) | 19.4/3.69 (mAh/cm2) | [110] | |
CoSe@NC | Nanofiber membrane | 1.2-1.5/69.19 | 200 (1 C) | 797/669.1 | [111] | |
VN/C@TCF | Triple-nanolayers | 1.5/N/A | 500 (1 C) | 978.1/661.2 | [112] |
Despite these achievements, challenges remain that must be addressed to enable the large-scale commercialization of Li-S batteries. Key limitations include: 1. Material and Cost Constraints: Molybdenum concentrate (MoS2) trades at ~20-25 USD/lb, while MoNx/MoCx synthesis requires additional nitridation/carbonization steps, increasing costs by 30%-50%; and 2. Scalability Barriers: Energy-intensive synthesis methods [e.g., high-temperature processing, chemical vapor deposition (CVD), sol-gel] hinder scalable production of MoCx/MoNx due to specialized equipment demands
First, the scalability and cost-effectiveness of Mo-based materials with precise nanostructures and heterostructures must be improved. The development of eco-friendly and economically viable synthesis methods, such as utilizing bio-based carbon sources or scalable templating approaches, will be essential. Secondly, the long-term stability of Mo-based catalysts under operating conditions, including their resistance to external factors and sulfur poisoning, requires further investigation. Doping strategies and surface engineering can be employed to enhance the durability of these materials. Thirdly, the interaction mechanisms between Mo-based materials and LiPSs must be better understood by performing advanced theoretical calculations and in situ characterization in order to gain insights into the optimization of the catalytic activity and adsorption capabilities.
Looking ahead, future research should focus on the design of multifunctional Mo-based composites by combining the catalytic benefits of MoCx and MoNx with the stability and conductivity of carbon architectures or other metallic compounds. Additionally, novel heterostructures, such as Mo-based materials integrated with transition metal sulfides, oxides, or selenides, may unfold new synergies. Finally, practical considerations, such as enhanced sulfur loadings, reduced electrolyte amount, and improved safety through dendrite-free lithium deposition[115], must be prioritized to bridge the gap between laboratory demonstrations and real-world applications.
On the other hand, replacing liquid electrolytes with solid-state electrolytes (SSEs), particularly sulfide-based SSEs in all-solid-state Li-S batteries (ASSLSBs), can physically eliminate polysulfide shuttle while mitigating safety risks associated with volatile and flammable solvents in conventional liquid electrolyte batteries[116]. However, the sulfur cathodes in ASSLSBs suffer from slow reaction kinetics and insufficient conductivity, necessitating the incorporation of substantial carbon additives and SSEs to enhance ionic/electronic transport around sulfur. Nevertheless, the resulting high interfacial contact area between SSEs and carbon induces non-negligible oxidative decomposition of SSEs during battery charging. Mo-based compounds demonstrate exceptional interfacial compatibility and stability with sulfides, serving as alternative sulfur hosts to effectively mitigate this issue[117,118]. Therefore, the application of MoCx/MoNx in ASSLSBs can become one of the research hotspots and challenges in the future LSB field.
By addressing these challenges and leveraging the unique properties of Mo-based materials, Li-S batteries can achieve the breakthroughs needed to become a competitive and sustainable solution for large-scale energy storage. The continued innovation in materials design, coupled with a deeper understanding of the underlying mechanisms, holds the potential to revolutionize the field of energy storage and significantly advance the transition to a green economy with carbon neutrality.
DECLARATIONS
Authors’ contributions
Framework design and manuscript writing: Fan, Q.
Manuscript review: Song, H.; Gao, B.; Wang, L.; Chu, P. K.
Administrative support, funding acquisition and supervision: Wang, L.; Chu, P. K.; Huo, K.
Availability of data and materials
Not applicable.
Financial support and sponsorship
This work was supported by the National Natural Science Foundation of China (Grant No. 22308109), Guangdong Basic and Applied Basic Research Foundation (Grant No. 2024A1515010678), Basic and Applied Basic Research Project of Guangzhou (Grant No. SL2023A04J00635), and City University of Hong Kong Donation Research Grants (DON-RMG 9229021 and 9229061).
Conflicts of interest
All authors declared that there are no conflicts of interest.
Ethical approval and consent to participate
Not applicable.
Consent for publication
Not applicable.
Copyright
© The Author(s) 2025.
REFERENCES
1. Khan, F. M. N. U.; Rasul, M. G.; Sayem, A.; Mandal, N. K. Design and optimization of lithium-ion battery as an efficient energy storage device for electric vehicles: a comprehensive review. J. Energy. Storage. 2023, 71, 108033.
2. Cheng, W.; Zhao, M.; Lai, Y.; et al. Recent advances in battery characterization using in situ XAFS, SAXS, XRD, and their combining techniques: from single scale to multiscale structure detection. Exploration 2024, 4, 20230056.
3. Nekahi, A.; Madikere, Raghunatha., Reddy., A. K.; Li, X.; Deng, S.; Zaghib, K. Rechargeable batteries for the electrification of society: past, present, and future. Electrochem. Energy. Rev. 2025, 8, 235.
4. Park, C. Y.; Kim, J.; Lim, W. G.; Lee, J. Toward maximum energy density enabled by anode-free lithium metal batteries: recent progress and perspective. Exploration 2024, 4, 20210255.
5. Huang, Z.; Jaumaux, P.; Sun, B.; et al. High-energy room-temperature sodium-sulfur and sodium-selenium batteries for sustainable energy storage. Electrochem. Energy. Rev. 2023, 6, 182.
6. Guo, Y.; Niu, Q.; Pei, F.; et al. Interface engineering toward stable lithium-sulfur batteries. Energy. Environ. Sci. 2024, 17, 1330-67.
7. Sharma, R.; Kumar, H.; Kumar, G.; et al. Progress and challenges in electrochemical energy storage devices: Fabrication, electrode material, and economic aspects. Chem. Eng. J. 2023, 468, 143706.
8. Liu, R.; Wei, Z.; Peng, L.; et al. Establishing reaction networks in the 16-electron sulfur reduction reaction. Nature 2024, 626, 98-104.
9. Wu, J.; Ye, T.; Wang, Y.; et al. Understanding the catalytic kinetics of polysulfide redox reactions on transition metal compounds in Li-S batteries. ACS. Nano. 2022, 16, 15734-59.
10. Li, J.; Niu, Z.; Guo, C.; Li, M.; Bao, W. Catalyzing the polysulfide conversion for promoting lithium sulfur battery performances: a review. J. Energy. Chem. 2021, 54, 434-51.
11. Li, G.; Wang, S.; Zhang, Y.; Li, M.; Chen, Z.; Lu, J. Revisiting the role of polysulfides in lithium-sulfur batteries. Adv. Mater. 2018, 30, e1705590.
12. Yu, J.; Pinto-Huguet, I.; Zhang, C. Y.; et al. Mechanistic insights and technical challenges in sulfur-based batteries: a comprehensive in situ/operando monitoring toolbox. ACS. Energy. Lett. 2024, 9, 6178-214.
13. Zhou, L.; Danilov, D. L.; Qiao, F.; et al. Sulfur reduction reaction in lithium-sulfur batteries: mechanisms, catalysts, and characterization. Adv. Energy. Mater. 2022, 12, 2202094.
14. Deng, R.; Wang, M.; Yu, H.; et al. Recent advances and applications toward emerging lithium-sulfur batteries: working principles and opportunities. Energy. Environ. Mater. 2022, 5, 777-99.
15. Yu, S.; Cai, W.; Chen, L.; Song, L.; Song, Y. Recent advances of metal phosphides for Li-S chemistry. J. Energy. Chem. 2021, 55, 533-48.
16. Hencz, L.; Chen, H.; Wu, Z.; et al. Highly branched amylopectin binder for sulfur cathodes with enhanced performance and longevity. Exploration 2022, 2, 20210131.
17. Zhai, P.; Peng, H.; Cheng, X.; et al. Scaled-up fabrication of porous-graphene-modified separators for high-capacity lithium-sulfur batteries. Energy. Storage. Mater. 2017, 7, 56-63.
18. Zhou, G.; Li, L.; Ma, C.; et al. A graphene foam electrode with high sulfur loading for flexible and high energy Li-S batteries. Nano. Energy. 2015, 11, 356-65.
19. He, G.; Evers, S.; Liang, X.; Cuisinier, M.; Garsuch, A.; Nazar, L. F. Tailoring porosity in carbon nanospheres for lithium-sulfur battery cathodes. ACS. Nano. 2013, 7, 10920-30.
20. Ruan, J.; Sun, H.; Song, Y.; et al. Constructing 1D/2D interwoven carbonous matrix to enable high-efficiency sulfur immobilization in Li-S battery. Energy. Mater. 2022, 1, 100018.
21. Wang, Q.; Zhao, H.; Li, B.; et al. MOF-derived Co9S8 nano-flower cluster array modified separator towards superior lithium sulfur battery. Chin. Chem. Lett. 2021, 32, 1157-60.
22. Tao, X.; Wang, J.; Liu, C.; et al. Balancing surface adsorption and diffusion of lithium-polysulfides on nonconductive oxides for lithium-sulfur battery design. Nat. Commun. 2016, 7, 11203.
23. He, J.; Chen, Y.; Manthiram, A. Vertical Co9S8 hollow nanowall arrays grown on a Celgard separator as a multifunctional polysulfide barrier for high-performance Li-S batteries. Energy. Environ. Sci. 2018, 11, 2560-8.
24. Liu, D.; Zhang, C.; Zhou, G.; et al. Catalytic effects in lithium-sulfur batteries: promoted sulfur transformation and reduced shuttle effect. Adv. Sci. 2018, 5, 1700270.
25. Zheng, Y.; Yi, Y.; Fan, M.; et al. A high-entropy metal oxide as chemical anchor of polysulfide for lithium-sulfur batteries. Energy. Storage. Mater. 2019, 23, 678-83.
26. Liu, X.; Huang, J. Q.; Zhang, Q.; Mai, L. Nanostructured metal oxides and sulfides for lithium-sulfur batteries. Adv. Mater. 2017, 29, 1601759.
27. Ye, X.; Wu, F.; Xue, Z.; et al. Accelerated polysulfide conversion by rationally designed NiS2-CoS2 heterostructure in lithium-sulfur batteries. Adv. Funct. Mater. 2025, 35, 2417776.
28. Huang, C.; Yu, J.; Zhang, C. Y.; et al. Electronic spin alignment within homologous NiS2/NiSe2 heterostructures to promote sulfur redox kinetics in lithium-sulfur batteries. Adv. Mater. 2024, 36, e2400810.
29. Zhao, H.; Wu, J.; Chen, T.; et al. Cobalt-doped molybdenum sulfide as an interlayer facilitates polysulfide conversion to obtain high-performance lithium-sulfur batteries. J. Energy. Storage. 2024, 101, 113903.
30. Ren, X.; Wu, H.; Guo, Y.; et al. The impact of oxygen content in O-doped MoS2 on the kinetics of polysulfide conversion in Li-S batteries. Small 2024, 20, e2312256.
31. Li, R.; Sun, H.; Chang, C.; Yao, Y.; Pu, X.; Mai, W. In situ induced cation-vacancies in metal sulfides as dynamic electrocatalyst accelerating polysulfides conversion for Li-S battery. J. Energy. Chem. 2022, 75, 74-82.
32. Yao, Y.; Chang, C.; Sun, H.; et al. Hollow Ni3Se4 with high tap density as a carbon-free sulfur immobilizer to realize high volumetric and gravimetric capacity for lithium-sulfur batteries. ACS. Appl. Mater. Interfaces. 2022, 14, 25267-77.
33. Cai, D.; Liu, B.; Zhu, D.; et al. Ultrafine Co3Se4 nanoparticles in nitrogen-doped 3D carbon matrix for high-stable and long-cycle-life lithium-sulfur batteries. Adv. Energy. Mater. 2020, 10, 1904273.
34. Mosavati, N.; Salley, S. O.; Ng, K. S. Characterization and electrochemical activities of nanostructured transition metal nitrides as cathode materials for lithium sulfur batteries. J. Power. Sources. 2017, 340, 210-6.
35. Jeong, T.; Choi, D. S.; Song, H.; et al. Heterogeneous catalysis for lithium-sulfur batteries: enhanced rate performance by promoting polysulfide fragmentations. ACS. Energy. Lett. 2017, 2, 327-33.
36. Zhang, L.; Chen, X.; Wan, F.; et al. Enhanced electrochemical kinetics and polysulfide traps of indium nitride for highly stable lithium-sulfur batteries. ACS. Nano. 2018, 12, 9578-86.
37. Weng, W.; Xiao, J.; Shen, Y.; Liang, X.; Lv, T.; Xiao, W. Molten salt electrochemical modulation of iron-carbon-nitrogen for lithium-sulfur batteries. Angew. Chem. Int. Ed. 2021, 60, 24905-9.
38. Wang, S.; Liu, X.; Duan, H.; Deng, Y.; Chen, G. Fe3C/Fe nanoparticles embedded in N-doped porous carbon nanosheets and graphene: a thin functional interlayer for PP separator to boost performance of Li-S batteries. Chem. Eng. J. 2021, 415, 129001.
39. Ye, Z.; Jiang, Y.; Qian, J.; et al. Exceptional adsorption and catalysis effects of hollow polyhedra/carbon nanotube confined CoP nanoparticles superstructures for enhanced lithium-sulfur batteries. Nano. Energy. 2019, 64, 103965.
40. Wu, Z.; Chen, S.; Wang, L.; et al. Implanting nickel and cobalt phosphide into well-defined carbon nanocages: a synergistic adsorption-electrocatalysis separator mediator for durable high-power Li-S batteries. Energy. Storage. Mater. 2021, 38, 381-8.
41. Wang, F.; Han, Y.; Xu, R.; et al. Establishing transition metal phosphides as effective sulfur hosts in lithium-sulfur batteries through the triple effect of “confinement-adsorption-catalysis”. Small 2023, 19, e2303599.
42. Susarla, S.; Puthirath, A. B.; Tsafack, T.; Salpekar, D.; Babu, G.; Ajayan, P. M. Atomic-level alloying of sulfur and selenium for advanced lithium batteries. ACS. Appl. Mater. Interfaces. 2020, 12, 1005-13.
43. Liu, Q.; Wu, Y.; Li, D.; et al. Dilute alloying to implant activation centers in nitride electrocatalysts for lithium-sulfur batteries. Adv. Mater. 2023, 35, e2209233.
44. Zhao, M.; Li, B. Q.; Peng, H. J.; Yuan, H.; Wei, J. Y.; Huang, J. Q. Lithium-sulfur batteries under lean electrolyte conditions: challenges and opportunities. Angew. Chem. Int. Ed. 2020, 59, 12636-52.
45. Xie, J.; Li, B. Q.; Peng, H. J.; et al. Implanting atomic cobalt within mesoporous carbon toward highly stable lithium-sulfur batteries. Adv. Mater. 2019, 31, e1903813.
46. Li, B.; Kong, L.; Zhao, C.; et al. Expediting redox kinetics of sulfur species by atomic-scale electrocatalysts in lithium-sulfur batteries. InfoMat 2019, 1, 533-41.
47. Zhang, Y.; Zhang, R.; Guo, Y.; Li, Y.; Li, K. A review on MoS2 structure, preparation, energy storage applications and challenges. J. Alloys. Compd. 2024, 998, 174916.
48. Cao, Y.; Lin, Y.; Wu, J.; et al. Two-dimensional MoS2 for Li-S batteries: structural design and electronic modulation. ChemSusChem 2020, 13, 1392-408.
49. Liu, Y.; Lin, Z.; Bettels, F.; et al. Molybdenum-based catalytic materials for Li-S batteries: strategies, mechanisms, and prospects. Adv. Energy. Sustain. Res. 2023, 4, 2200145.
50. Liu, Y.; Cui, C.; Liu, Y.; Liu, W.; Wei, J. Application of MoS2 in the cathode of lithium sulfur batteries. RSC. Adv. 2020, 10, 7384-95.
51. Hussain, I.; Amara, U.; Bibi, F.; et al. Mo-based MXenes: synthesis, properties, and applications. Adv. Colloid. Interface. Sci. 2024, 324, 103077.
52. Hua, W.; Sun, H.; Xu, F.; Wang, J. A review and perspective on molybdenum-based electrocatalysts for hydrogen evolution reaction. Rare. Met. 2020, 39, 335-51.
53. Miao, M.; Pan, J.; He, T.; Yan, Y.; Xia, B. Y.; Wang, X. Molybdenum carbide-based electrocatalysts for hydrogen evolution reaction. Chem. A. Eur. J. 2017, 23, 10947-61.
54. Wan, C.; Regmi, Y. N.; Leonard, B. M. Multiple phases of molybdenum carbide as electrocatalysts for the hydrogen evolution reaction. Angew. Chem. Int. Ed. 2014, 53, 6407-10.
55. Ramqvist, L.; Hamrin, K.; Johansson, G.; Fahlman, A.; Nordling, C. Charge transfer in transition metal carbides and related compounds studied by ESCA. J. Phys. Chem. Solids. 1969, 30, 1835-47.
56. Jansen, S. A.; Hoffmann, R. Surface chemistry of transition metal carbides: a theoretical analysis. Surf. Sci. 1988, 197, 474-508.
57. Zhang, Y.; Wang, Y.; Guo, C.; Wang, Y. Molybdenum carbide-based photocatalysts: synthesis, functionalization, and applications. Langmuir 2022, 38, 12739-56.
58. Zhong, Y.; Xia, X.; Shi, F.; Zhan, J.; Tu, J.; Fan, H. J. Transition metal carbides and nitrides in energy storage and conversion. Adv. Sci. 2016, 3, 1500286.
59. Xie, J.; Li, S.; Zhang, X.; et al. Atomically-thin molybdenum nitride nanosheets with exposed active surface sites for efficient hydrogen evolution. Chem. Sci. 2014, 5, 4615-20.
60. Sun, M.; Wang, Z.; Li, X.; et al. Rational understanding of the catalytic mechanism of molybdenum carbide in polysulfide conversion in lithium-sulfur batteries. J. Mater. Chem. A. 2020, 8, 11818-23.
61. Razaq, R.; Sun, D.; Xin, Y.; et al. Enhanced kinetics of polysulfide redox reactions on Mo2C/CNT in lithium-sulfur batteries. Nanotechnology 2018, 29, 295401.
62. Qian, J.; Xing, Y.; Yang, Y.; et al. Enhanced electrochemical kinetics with highly dispersed conductive and electrocatalytic mediators for lithium-sulfur batteries. Adv. Mater. 2021, 33, e2100810.
63. Wang, L.; He, L.; Cheng, Y.; et al. P-doped Mo2C nanoparticles embedded on carbon nanofibers as an efficient electrocatalyst for Li-S batteries. Chem. Eng. J. 2024, 490, 151530.
64. Chen, K.; Zhu, Y.; Huang, Z.; et al. Strengthened d-p orbital hybridization on metastable cubic Mo2C for highly stable lithium-sulfur batteries. ACS. Nano. 2024, 18, 34791-802.
65. Li, J.; Shi, K.; Pan, J.; et al. Designing electrochemical nanoreactors to accelerate Li2S1/2 three-dimensional growth process and generating more Li2S for advanced Li-S batteries. Renewables 2023, 1, 341-52.
66. Yang, J.; Zhao, S.; Lu, Y.; Zeng, X.; Lv, W.; Cao, G. In-situ topochemical nitridation derivative MoO2-Mo2N binary nanobelts as multifunctional interlayer for fast-kinetic Li-Sulfur batteries. Nano. Energy. 2020, 68, 104356.
67. Ma, Y.; Chang, L.; Yi, D.; et al. Synergetic effect of block and catalysis on polysulfides by functionalized bilayer modification on the separator for lithium-sulfur batteries. Energy. Mater. 2024, 4, 400059.
68. Yu, B.; Chen, D.; Wang, Z.; et al. Mo2C quantum dots@graphene functionalized separator toward high-current-density lithium metal anodes for ultrastable Li-S batteries. Chem. Eng. J. 2020, 399, 125837.
69. Shi, H.; Sun, Z.; Lv, W.; et al. Necklace-like MoC sulfiphilic sites embedded in interconnected carbon networks for Li-S batteries with high sulfur loading. J. Mater. Chem. A. 2019, 7, 11298-304.
70. Ji, Y.; Zhang, J.; Yang, N.; et al. MoC nanoparticles decorated carbon nanofibers loaded with Li2S as high-performance lithium sulfur battery cathodes. Appl. Surf. Sci. 2025, 679, 161263.
71. Li, H.; Zheng, W.; Wu, H.; Fang, Y.; Li, L.; Yuan, W. Ultra-dispersed α-MoC1-x embedded in a plum-like N-doped carbon framework as a synergistic adsorption-electrocatalysis interlayer for high-performance Li-S batteries. Small 2024, 20, e2306140.
72. Zhang, H.; Jin, H.; Yang, Y.; et al. Understanding the synergetic interaction within α-MoC/β-Mo2C heterostructured electrocatalyst. J. Energy. Chem. 2019, 35, 66-70.
73. Han, K.; Guo, D.; Li, M.; et al. Mixed valence Mo2C-MoC/C catalyst enhances polysulfides conversion kinetics in lithium-sulfur batteries. Appl. Surf. Sci. 2024, 663, 160138.
74. Liu, X.; Wang, J.; Wang, W.; et al. Interfacial synergy in Mo2C/MoC heterostructure promoting sequential polysulfide conversion in high-performance lithium-sulfur battery. Small 2024, 20, e2307902.
75. Jiang, G.; Xu, F.; Yang, S.; Wu, J.; Wei, B.; Wang, H. Mesoporous, conductive molybdenum nitride as efficient sulfur hosts for high-performance lithium-sulfur batteries. J. Power. Sources. 2018, 395, 77-84.
76. Ma, F.; Srinivas, K.; Zhang, X.; et al. Mo2N Quantum dots decorated N-doped graphene nanosheets as dual-functional interlayer for dendrite-free and shuttle-free lithium-sulfur batteries. Adv. Funct. Mater. 2022, 32, 2206113.
77. Yang, M.; Liu, P.; Qu, Z.; et al. Nitrogen-vacancy-regulated Mo2N quantum dots electrocatalyst enables fast polysulfides redox for high-energy-density lithium-sulfur batteries. Nano. Energy. 2022, 104, 107922.
78. Cheng, M.; Xing, Z.; Yan, R.; et al. Oxygen-modulated metal nitride clusters with moderate binding ability to insoluble Li2Sx for reversible polysulfide electrocatalysis. InfoMat 2023, 5, e12387.
79. Liu, Z.; Lian, R.; Wu, Z.; et al. Ordered dual-channel carbon embedded with molybdenum nitride catalytically induced high-performance lithium-sulfur battery. Chem. Eng. J. 2022, 431, 134163.
80. Tan, T.; Chen, N.; Wang, Z.; et al. Thorn-like carbon nanofibers combined with molybdenum nitride nanosheets as a modified separator coating: an efficient chemical anchor and catalyst for Li-S batteries. ACS. Appl. Energy. Mater. 2022, 5, 6654-62.
81. Li, R.; Peng, H.; Wu, Q.; et al. Sandwich-like catalyst-carbon-catalyst trilayer structure as a compact 2D host for highly stable lithium-sulfur batteries. Angew. Chem. Int. Ed. 2020, 59, 12129-38.
82. Liu, Y.; Xu, J.; Cao, Y.; et al. Promoting polysulfide redox kinetics by tuning the non-metallic p-band of Mo-based compounds. J. Mater. Chem. A. 2022, 10, 11477-87.
83. Kong, Y.; Wang, L.; Mamoor, M.; et al. Co/Mon invigorated bilateral kinetics modulation for advanced lithium-sulfur batteries. Adv. Mater. 2024, 36, e2310143.
84. Dewangan, K.; Patil, S. S.; Joag, D. S.; More, M. A.; Gajbhiye, N. S. Topotactical nitridation of α-MoO3 fibers to γ-Mo2N fibers and its field emission properties. J. Phys. Chem. C. 2010, 114, 14710-5.
85. Machon, D.; Daisenberger, D.; Soignard, E.; et al. High pressure - high temperature studies and reactivity of γ-Mo2N and δ-MoN. Phys. Status. Solidi. 2006, 203, 831-6.
86. Liu, S.; Xiao, J.; Lu, X. F.; Wang, J.; Wang, X.; Lou, X. W. D. Efficient electrochemical reduction of CO2 to HCOOH over Sub-2 nm SnO2 quantum wires with exposed grain boundaries. Angew. Chem. Int. Ed. 2019, 58, 8499-503.
87. Wang, C.; Wang, S.; He, Y.; et al. Combining fast Li-ion battery cycling with large volumetric energy density: grain boundary induced high electronic and ionic conductivity in Li4Ti5O12 spheres of densely packed nanocrystallites. Chem. Mater. 2015, 27, 5647-56.
88. Yang, J.; Cai, D.; Lin, Q.; et al. Regulating the Li2S deposition by grain boundaries in metal nitrides for stable lithium-sulfur batteries. Nano. Energy. 2022, 91, 106669.
89. Niu, S.; Zhang, S.; Shi, R.; et al. Freestanding agaric-like molybdenum carbide/graphene/N-doped carbon foam as effective polysulfide anchor and catalyst for high performance lithium sulfur batteries. Energy. Storage. Mater. 2020, 33, 73-81.
90. Wang, P.; Li, N.; Zhang, Z.; et al. Synergetic enhancement of polysulfide chemisorption and electrocatalysis over bicontinuous MoN@N-rich carbon porous nano-octahedra for Li-S batteries. J. Mater. Chem. A. 2019, 7, 21934-43.
91. Chen, G.; Li, Y.; Zhong, W.; et al. MOFs-derived porous Mo2C-C nano-octahedrons enable high-performance lithium-sulfur batteries. Energy. Storage. Mater. 2020, 25, 547-54.
92. Wang, Q.; Qin, B.; Jiang, Q.; et al. Highly dispersed conductive and electrocatalytic mediators enabling rapid polysulfides conversion for lithium sulfur batteries. Chem. Eng. J. 2023, 476, 146865.
93. Huang, S.; Wang, Z.; Von, Lim., Y.; et al. Recent advances in heterostructure engineering for lithium-sulfur batteries. Adv. Energy. Mater. 2021, 11, 2003689.
94. Lee, H.; Nam, H.; Moon, J. H. Seamless integration of nanoscale crystalline-amorphous MoO3 domains for high-performance lithium-sulfur batteries. Energy. Storage. Mater. 2024, 70, 103551.
95. Jiao, X.; Hu, J.; Zuo, Y.; Qi, J.; Yan, W.; Zhang, J. Self-recovery catalysts of ZnIn2S4@In2O3 heterostructures with multiple catalytic centers for cascade catalysis in lithium-sulfur battery. Nano. Energy. 2024, 119, 109078.
96. Zhao, M.; Tan, P.; Cai, D.; et al. Customizing component regulated dense heterointerfaces for crafting robust lithium-sulfur batteries. Adv. Funct. Mater. 2023, 33, 2211505.
97. Zhang, W.; Li, H.; Tao, R.; et al. In-situ constructing hetero-structured Mo2C-Mo2N embedded in carbon nanosheet as an efficient separator modifier for high-performance lithium-sulfur batteries. Chem. Eng. J. 2023, 475, 146133.
98. Li, X. H.; Antonietti, M. Metal nanoparticles at mesoporous N-doped carbons and carbon nitrides: functional Mott-Schottky heterojunctions for catalysis. Chem. Soc. Rev. 2013, 42, 6593-604.
99. Wang, Y.; Zhang, R.; Sun, Z.; et al. Spontaneously formed mott-schottky electrocatalyst for lithium-sulfur batteries. Adv. Mater. Inter. 2020, 7, 1902092.
100. Li, X.; Zuo, Y.; Zhang, Y.; et al. Controllable sulfurization of MXenes to in-plane multi-heterostructures for efficient sulfur redox kinetics. Adv. Energy. Mater. 2024, 14, 2303389.
101. Du, S.; Yu, Y.; Liu, X.; et al. Heterostructure Mo2C/α-MoO3/G catalyst based heterogeneous catalysis/deposition mechanism for high-performance Li-S battery. Chem. Eng. J. 2024, 500, 157002.
102. Zhang, K.; Zhao, Z.; Chen, H.; et al. A review of advances in heterostructured catalysts for Li-S batteries: structural design and mechanism analysis. Small 2025, 21, e2409674.
103. Chen, L.; Cao, G.; Li, Y.; et al. A review on engineering transition metal compound catalysts to accelerate the redox kinetics of sulfur cathodes for lithium-sulfur batteries. Nanomicro. Lett. 2024, 16, 97.
104. Yan, L.; Luo, N.; Kong, W.; et al. Enhanced performance of lithium-sulfur batteries with an ultrathin and lightweight MoS2/carbon nanotube interlayer. J. Power. Sources. 2018, 389, 169-77.
105. Imtiaz, S.; Ali, Zafar., Z.; Razaq, R.; et al. Electrocatalysis on separator modified by molybdenum trioxide nanobelts for lithium-sulfur batteries. Adv. Mater. Inter. 2018, 5, 1800243.
106. Wang, L.; Li, X.; Zhang, Y.; et al. Subnanometer MoP clusters confined in mesoporous carbon (CMK-3) as superior electrocatalytic sulfur hosts for high-performance lithium-sulfur batteries. Chem. Eng. J. 2022, 446, 137050.
107. Jiang, Y.; Du, M.; Geng, P.; Sun, B.; Zhu, R.; Pang, H. CoO/MoO3@Nitrogen-doped carbon hollow heterostructures for efficient polysulfide immobilization and enhanced ion transport in lithium-sulfur batteries. J. Colloid. Interface. Sci. 2024, 664, 617-25.
108. Song, M.; Liu, Y.; Wang, X.; et al. Atomic substitution engineering-induced domino synergistic catalysis in Li-S batteries. Chem. Eng. J. 2024, 502, 157926.
109. Liu, Y.; Meng, Q.; Yang, R.; et al. Anchoring polysulfides with ternary Fe3O4/graphitic carbon/porous carbon fiber hierarchical structures for high-rate lithium-sulfur batteries. J. Energy. Storage. 2025, 105, 114591.
110. Lu, Y.; Qin, J.; Shen, T.; et al. Hypercrosslinked polymerization enabled N-doped carbon confined Fe2O3 facilitating Li polysulfides interface conversion for Li-S batteries. Adv. Energy. Mater. 2021, 11, 2101780.
111. Jiang, X.; Zhang, S.; Zou, B.; et al. Electrospun CoSe@NC nanofiber membrane as an effective polysulfides adsorption-catalysis interlayer for Li-S batteries. Chem. Eng. J. 2022, 430, 131911.
112. Liu, G.; Zeng, Q.; Sui, X.; et al. Modulating the d-p orbital coupling of manganese chalcogenides for efficient polysulfides conversion in lithium-sulfur batteries. J. Power. Sources. 2022, 552, 232244.
113. Zhang, W.; Liu, J.; Cai, W.; et al. Engineering d-p orbital hybridization through regulation of interband energy separation for durable aqueous Zn//VO2(B) batteries. Chem. Eng. J. 2023, 464, 142711.
114. Yu, J.; Yong, X.; Lu, S. p-d orbital hybridization engineered single-atom catalyst for electrocatalytic ammonia synthesis. Energy. Environ. Mater. 2024, 7, e12587.
115. Yu, Z.; Gan, C.; Mijailovic, A. S.; et al. Lithium dendrite deflection at mixed ionic-electronic conducting interlayers in solid electrolytes. Adv. Energy. Mater. 2025, 15, 2403179.
116. Shen, K.; Shi, W.; Song, H.; et al. Solid catholyte with regulated interphase redox for all-solid-state lithium-sulfur batteries. Adv. Mater. 2025, 37, e2417171.
117. George, C.; Morris, A. J.; Modarres, M. H.; De, Volder., M. Structural evolution of electrochemically lithiated MoS2 nanosheets and the role of carbon additive in Li-ion batteries. Chem. Mater. 2016, 28, 7304-10.
Cite This Article

How to Cite
Download Citation
Export Citation File:
Type of Import
Tips on Downloading Citation
Citation Manager File Format
Type of Import
Direct Import: When the Direct Import option is selected (the default state), a dialogue box will give you the option to Save or Open the downloaded citation data. Choosing Open will either launch your citation manager or give you a choice of applications with which to use the metadata. The Save option saves the file locally for later use.
Indirect Import: When the Indirect Import option is selected, the metadata is displayed and may be copied and pasted as needed.
About This Article
Copyright
Data & Comments
Data
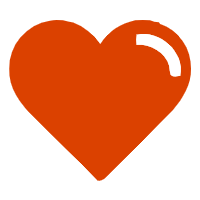
Comments
Comments must be written in English. Spam, offensive content, impersonation, and private information will not be permitted. If any comment is reported and identified as inappropriate content by OAE staff, the comment will be removed without notice. If you have any queries or need any help, please contact us at support@oaepublish.com.