The impact of biomaterial characteristics on macrophage phenotypes in tissue engineering: a review
Abstract
Macrophages are highly plastic cells central to pathogen removal, tissue regeneration, and inflammation, making them key targets in biomaterial design for improved clinical outcomes. Foreign body responses (FBRs) to implanted biomaterials often involve excessive macrophage-mediated inflammation, leading to fibrotic encapsulation, infection, and implant failure. Advances in tissue engineering demonstrate that macrophage polarization - the transition from pro-inflammatory M1 to anti-inflammatory M2 phenotypes - can be influenced by biomaterial properties to mitigate these responses and enhance regeneration. This review synthesizes the relationship between biomaterial properties, such as surface chemistry, structure, and stiffness, and their ability to modulate macrophage behavior. Key innovations, including tailored scaffold architectures, bioactive coatings, and cytokine delivery systems, have shown promise in guiding macrophage polarization for improved bone, soft tissue, and head and neck reconstruction outcomes. Strategies like hydrogels and nanostructured materials enable spatially and temporally controlled macrophage modulation, mimicking native extracellular matrix dynamics, mitigating chronic inflammation, and accelerating vascularization, extracellular matrix remodeling, and tissue integration. By integrating recent findings, this review provides a framework for designing biomaterials that actively modulate macrophage activity to overcome FBR and enhance healing. It identifies critical gaps, such as understanding macrophage-stromal interactions, developing personalized biomaterial designs to address patient variability, and leveraging advanced technologies like artificial intelligence in scaffold optimization. These insights advance the development of biomaterials that restore tissue function and address unmet clinical needs in regenerative medicine.
Keywords
INTRODUCTION
Since the early 2000s, biomaterials such as drug delivery systems, biosensors, and tissue scaffolds have expanded to meet various clinical needs[1]. Biomaterials are typically made of metals, polymers, ceramics, and other composite forms[2]. Successful implantation depends on the material’s physical, chemical, and mechanical properties and the foreign body response[3]. Macrophages modulate this response, driving inflammation or promoting healing.
Tissue engineering has improved outcomes in reconstructive surgery, organ failure, and major tissue damage. A significant development is biomaterial scaffolds, which mimic the extracellular matrix (ECM) to promote cell adhesion, migration, and tissue repair. For example, microRNA-integrated scaffolds regulate cellular behavior and accelerate regeneration[4]. Similarly, integrating bioprinting and artificial intelligence shows promise for patient-specific implants with optimized biological properties[5].
Implanted biomaterials trigger an immune response starting with the adsorption of proteins onto the biomaterial surface, which recruits macrophages and other immune cells. Over time, macrophages can either resolve inflammation, promote healing, or cause fibrous encapsulation of the biomaterial. Therefore, modulating macrophage-biomaterial interactions is essential to improving implant integration.
Despite advancements in biomaterial research, challenges remain, including inadequate vascularization and patient-specific immune responses, which limit implant success[6]. Addressing these gaps requires interdisciplinary collaboration to improve the clinical translation of emerging technologies[7].
Macrophages are important regulators of wound healing and immune response, with their origin, activation state, and function influencing outcomes. Macrophages arise from tissue-resident populations seeded during development or circulating monocytes recruited after injury. Once in the tissue, monocytes differentiate and polarize based on local signals. Activated macrophages occur along a spectrum, with macrophages classically divided into the pro-inflammatory M1 phenotype, which responds to pathogens and mediates early inflammation, and the anti-inflammatory M2 phenotype, which promotes tissue repair and remodeling. This polarization is driven by cytokines, with lipopolysaccharide (LPS) and interferon-gamma (IFN-γ) for M1 polarization and interleukin-4 (IL-4) and interleukin-13 (IL-13) for M2 [Figure 1]. Functionally, M1 macrophages produce reactive oxygen species (ROS) and inflammatory cytokines, while M2 macrophages release transforming growth factor-beta (TGF-β) and interleukin-10 (IL-10) to facilitate ECM remodeling and tissue regeneration.
Figure 1. Macrophage polarization and biomaterial influence. The schematic illustrates the polarization of M0 macrophages into M1 and M2 phenotypes in response to distinct biochemical and environmental cues. M1 macrophages, driven by pro-inflammatory stimuli such as IFN-γ, TNF-α, GM-CSF, and LPS, are characterized by the expression of markers including iNOS, CD86, and CD80 and the secretion of inflammatory cytokines (e.g., TNF-α, IL-1β, IL-6). In contrast, M2 macrophages are induced by anti-inflammatory signals such as IL-4, IL-10, IL-13, and TGF-β, expressing markers like CD206, CD209, and FIZZ1, and secreting cytokines (e.g., IL-10, TGF-β) that promote tissue repair and immunomodulation. Engineered biomaterials modulate macrophage polarization: porous scaffolds, carbon nanotubes, and gold nanoparticles enhance M1 polarization, while hydrogels, lipid nanoparticles, micelles, and polymeric nanoparticles promote M2 polarization. Figure created in https://www.biorender.com/ and adapted from Yao et al.[8,9]. IFN-γ: Interferon-gamma; TNF-α: tumor necrosis factor-alpha; GM-CSF: granulocyte-macrophage colony-stimulating factor; LPS: lipopolysaccharide; iNOS: inducible nitric oxide synthase; TGF-β: transforming growth factor-beta; FIZZ1: found in inflammatory zone 1.
While Figure 1 illustrates the dichotomous classification of macrophages into M1 and M2 phenotypes, macrophage activation exists along a continuum of functional states. Single-cell RNA sequencing (scRNA-seq) has been instrumental in uncovering this heterogeneity in tissue repair contexts. In skin wound healing, scRNA-seq identified eight distinct monocyte/macrophage clusters with transcriptional profiles corresponding to early pro-inflammatory, late-stage pro-healing, and antigen-presenting phenotypes, highlighting dynamic state transitions throughout the healing process[10]. Similarly, analysis of infarcted hearts revealed a complex repertoire of macrophage subsets, including Trem2hi macrophages that emerge in the late phase post-myocardial infarction, contributing to anti-inflammatory signaling and tissue remodeling[11]. Macrophage populations involved in efferocytosis also display diverse transcriptional signatures, suggesting specialized roles in clearing apoptotic cells and promoting tissue repair[12]. Moreover, the integration of scRNA-seq data across models of atherosclerosis has identified heterogeneous macrophage phenotypes with distinct functions, ranging from inflammatory to lipid-handling and repair-associated states, further underscoring the limitations of the M1/M2 paradigm[13]. Therefore, to fully appreciate how biomaterials modulate macrophage activity, it is necessary to consider the full spectrum of macrophage heterogeneity and their dynamic responses to environmental cues beyond the M1/M2 framework.
In recent decades, the role of macrophages as regulators of wound healing and the specified functions of their polarized states (M1/M2) have made them the target of therapies designed to combat a variety of pathological states. Significantly, the acceptance or rejection of biomaterials such as implants, tissue grafts, and drug delivery devices, among others, largely depends on macrophage phenotype, underscoring their crucial role in the success of various medical devices and offering an avenue of interest for future classes of therapeutics.
Given the role of macrophages in wound healing and tissue regeneration, understanding how biomaterial properties influence their polarization is critical to improving clinical outcomes. This narrative review synthesizes recent findings on the impact of biomaterial characteristics - including structure, surface chemistry, and molecular modifications - on macrophage polarization, focusing on applications in tissue engineering. Key studies were identified through a targeted literature review, emphasizing research from the last decade that provides insights into the mechanisms by which biomaterials modulate macrophage behavior. The following sections discuss the inflammatory reaction and macrophage polarization, the influence of specific biomaterial properties, and applications in tissue engineering, particularly emphasizing bone regeneration, soft tissue repair, and head and neck reconstruction.
MACROPHAGE POLARIZATION IN WOUND HEALING PHASES
Macrophages play an essential role in all three phases of wound healing - inflammation, proliferation, and remodeling - through diverse phenotypes regulated by cytokine and mechanical cues. In the early inflammatory phase, macrophages adopt a pro-inflammatory (M1) phenotype driven by mediators such as LPS and IFN-γ [Figure 1]. M1 macrophages secrete ROS and phagocytose foreign materials for lysosomal degradation. In the later stages of healing, cytokines like IL-4 and IL-13 drive the transition to the anti-inflammatory M2 phenotype. M2 macrophages release mediators such as TGF-β and IL-10, facilitating ECM breakdown, cell migration, and tissue remodeling[14]. This critical shift from the M1 to M2 phenotype marks the transition from inflammation to tissue resolution, enabling repair and regeneration. However, in chronic wounds, such as arterial ulcers in diabetic patients or venous ulcers caused by poor circulation, the persistence of the M1 phenotype leads to prolonged inflammation and delayed healing. Similar M1 dominance is observed in implant rejection[15]. Conversely, excessive M2 activation can cause fibrosis and excessive scar formation, as shown in studies linking M2 depletion to reduced scarring[16]. Optimizing biomaterial properties (such as form, architecture, stiffness, and surface chemistry) can help regulate cytokine release and encourage the transition to M2 macrophages. Optimizing biomaterial properties - such as architecture, stiffness, and surface chemistry - can regulate cytokine release and promote the transition to the M2 phenotype, thereby improving tissue healing.
When exposed to an inflammatory stimulus, macrophages adopt the M1 phenotype and release reactive species and inflammatory cytokines to eliminate pathogens. However, shifts in the surrounding microenvironment can influence macrophage behavior, either facilitating or disrupting the transition from the pro-inflammatory M1 state to the anti-inflammatory M2 state[17]. In the early stages of regular tissue repair, macrophages polarize to the M1 phenotype (approximately 1-3 days) and transition to the M2 phenotype in the later stages (around 4-7 days). However, prolonged dominance of M1 macrophages can lead to excessive inflammation, which hinders tissue regeneration. M1 macrophages are known for their high expression of VEGF, promoting angiogenesis, while M2 macrophages primarily express PDGF-BB, stabilizing vascular growth. Since vascularization is essential for tissue repair, maintaining mild and regulated inflammation is crucial; therefore, a timely switch from M1 to M2 macrophages is necessary for successful tissue regeneration[18].
Molecular mechanisms and metabolic regulation of macrophage polarization
Inflammation, an inevitable consequence of implantation, is a critical factor in determining the clinical success of implants. Following implantation, immune cells, particularly monocytes and macrophages, migrate to the site and initiate a localized inflammatory response[19]. Although inflammation is essential for tissue regeneration, excessive or prolonged inflammatory responses can impair healing and result in fibrotic capsule formation around the implant, which may be tolerable in specific contexts but catastrophic in others, such as heart valve replacement[20]. Consequently, the immune response’s type and duration significantly impact tissue healing, determining whether the outcome is complete restoration or scarring and fibrosis. Biomaterials can help promote regeneration by modulating immune activity when implanted as temporary scaffolds.
Upon implantation, macrophages are recruited in their M0 state, and afterward, M1 macrophages initiate an inflammatory response to remove pathogens. However, prolonged M1 activity (over 3-4 days) can trigger FBR, which leads to fibrous encapsulation and potential implant failure. The immune response to implanted biomaterials progresses through initial, acute, and chronic stages. In the acute phase, macrophages are polarized to the M1 phenotype, where they release pro-inflammatory cytokines like TNF-α and IL-1β to initiate defense mechanisms against potential pathogens and promote tissue inflammation. This stage is crucial for immediate tissue protection but can be detrimental if sustained. Thus, biomaterials combined with cytokines like IL-4 and IFN-γ may help regulate this transition by promoting macrophage polarization.
In addition to IL-4, IL-10 is critical in promoting M2 macrophage polarization by activating the STAT3 pathway, which enhances anti-inflammatory responses and suppresses pro-inflammatory cytokines such as TNF-α and IL-1β[21,22]. IL-13, similar to IL-4, drives M2 polarization through STAT6 activation, while TGF-β supports ECM deposition and tissue repair via the SMAD2/3 pathway[23]. The molecular mechanisms underlying this transition are driven by IL-4-mediated activation of STAT6, which promotes the transcription of M2-associated genes such as arginase-1 (Arg1), mannose receptor (CD206), and resistin-like molecule alpha (Fizz1)[24]. STAT6 activation also facilitates chromatin remodeling to recruit retinoid X receptor (RXR) and peroxisome proliferator-activated receptor gamma (PPARγ), which regulate lipid metabolism critical for M2 polarization[25].
Macrophage polarization is tightly linked to metabolic reprogramming. M1 macrophages exhibit a metabolic shift toward aerobic glycolysis (Warburg effect), resulting in elevated production of ROS and nitric oxide (NO) via inducible nitric oxide synthase (iNOS). In parallel, disrupted tricarboxylic acid (TCA) cycle activity leads to succinate accumulation, which stabilizes hypoxia-inducible factor 1-alpha (HIF-1α) and amplifies IL-1β expression[26]. In contrast, M2 macrophages rely on oxidative phosphorylation (OXPHOS) and fatty acid oxidation (FAO) to sustain anti-inflammatory responses and tissue remodeling[26].
Synergistic metabolic pathways coordinate these phenotypic shifts. The mTORC1-HIF-1α axis enhances glycolytic flux and inflammatory cytokine production in M1 macrophages, while mTORC2-IRF4 signaling promotes lipid metabolism and OXPHOS to drive M2 polarization[26]. Additionally, AMPK activation suppresses mTORC1 and enhances FAO, reinforcing the M2 phenotype[26]. These metabolic signals converge on transcription factors such as PPARγ and STAT6, integrating energy metabolism with polarization programs[24,25].
Therefore, the sustained release of IL-4 from scaffolds encourages the shift from the pro-inflammatory M1 phenotype to the anti-inflammatory M2 phenotype, which is key to resolving inflammation and promoting tissue repair. For instance, scaffolds designed for bone regeneration incorporating IL-4 through biotin-streptavidin binding have demonstrated a sustained release over six days, effectively promoting the M1-to-M2 macrophage transition and enhancing vascularization in murine models[27]. TGF-β signaling further supports this transition through the SMAD2/3 pathway, promoting ECM remodeling and anti-inflammatory responses[28].
On the other hand, if inflammation is not adequately regulated, the chronic phase will continue, where prolonged activation of M1 macrophages leads to fibrous encapsulation and impaired biomaterial integration, hindering tissue regeneration. This chronic inflammation is typically characterized by a sustained foreign body response, commonly seen in cases involving metal plates for head and neck reconstruction, the formation of fistulas, and the extrusion of plates through the skin [Figure 2]. However, by controlling the polarization of macrophages through tailored biomaterial designs, the immune response can be guided from an inflammatory M1 state to a tissue-healing M2 state. This accelerates the resolution of inflammation and supports the chronic healing phase by enhancing tissue remodeling and integrating the implanted material. Overall, it can be said that modulating macrophage phenotype polarization across these stages can optimize the biomaterial’s effectiveness and promote better clinical outcomes.
Figure 2. Host immune response to biomaterial scaffold implantation. This schematic illustrates the sequential steps involved in the interaction between host immune cells and an implanted biomaterial scaffold, emphasizing the role of macrophages in tissue integration and repair. (1) The implantation of the biomaterial scaffold initiates the host immune response; (2) Adsorption of host proteins onto the biomaterial surface facilitates immune cell recognition; (3) Monocytes are recruited to the site, differentiate into M0 macrophages under the influence of M-CSF, and infiltrate the scaffold to initiate innate immune responses; (4) M1 macrophages release pro-inflammatory cytokines, recruiting additional immune cells, progenitors, and stem cells to the site; (5) M2 macrophages promote ECM remodeling by secreting anti-inflammatory cytokines and growth factors, facilitating tissue repair; (6) M2 macrophages drive tissue regeneration, vascular integration, and scaffold-host tissue compatibility. Figure created in https://www.biorender.com/ and adapted from Gaharwar et al.[9,29]. ECM: Extracellular matrix; M-CSF: macrophage colony-stimulating factor.
BIOMATERIAL PROPERTIES INFLUENCING MACROPHAGE POLARIZATION
Structural properties
Scaffold design includes porosity, surface area, stiffness, and coat materials. Studies have shown that these characteristics of biomaterials contribute to the inflammatory response and polarization of macrophages. Several studies have shown that the pore size of biomaterials can alter macrophage polarization. Liu et al., for instance, demonstrated that in 2D in vitro studies, smaller pore sizes reduce inflammatory response by promoting M2 macrophage polarization. The authors used a microporous annealed particle (MAP) hydrogel scaffold seeded with bone marrow-derived macrophages (BMDMs) under static culture conditions, where spatial confinement played a critical role in modulating cell morphology[30].
In contrast, larger pores increase the inflammatory response and decrease M2 macrophage polarization. Smaller pores induce a more spherical shape of the macrophages, which corresponds with M2 polarization[30]. Macrophages in smaller pores have lower expression of inflammatory cytokines such as IL-6 and TNF than those in larger pores[30]. In comparison with these findings, Wang et al. evaluated scaffolds with three different pore sizes and reported that larger pore sizes led to lower TNF-α expression and higher IL-10 expression, driving M2 macrophage polarization[31]. In this study, Wang et al. used 3D-printed polycaprolactone (PCL) scaffolds in a murine calvarial bone defect model. The in vivo nature of this model introduced dynamic factors, including mechanical loading, vascular infiltration, and cell migration, which likely contributed to the shift in macrophage behavior toward an M2 phenotype with larger pores. This cytokine profile also enhanced vascular ingrowth and bone regeneration, demonstrating the utility of larger pores in supporting bone healing applications[31].
In another study, using PCL/polyethylene glycol/hydroxyapatite scaffolds, Li et al. found that scaffolds with larger pore sizes reduced the foreign body response and induced more M2 macrophage infiltration, vascular ingrowth, and new bone formation[32]. Researchers in this study also performed in vivo implantation in a rat calvarial bone defect model, emphasizing the interplay between scaffold architecture and bone-specific immune responses, where larger pores improved cellular infiltration and angiogenesis necessary for osseointegration.
Research on hydroxyapatite particle size further underscores its role in macrophage polarization, with nano-scale HA particles inducing an anti-inflammatory M2 phenotype via IL-10-dependent pathways. In contrast, larger, micron-scale particles elicited pro-inflammatory responses[33].
Differences in biomaterial composition, cell sources, and experimental models can explain these divergent outcomes. In the 2D hydrogel model, Liu et al. used BMDMs in a static wound-healing-like environment, favoring M2 polarization due to softer matrix stiffness and confinement effects[30]. In contrast, thermoplastic scaffolds were used in vivo within bone tissue[31,32], where larger pores facilitated macrophage infiltration and promoted M2 polarization via pro-angiogenic signaling. This suggests that stiffer materials and load-bearing applications, such as bone regeneration, benefit from larger pores. In contrast, smaller pores may be advantageous in soft tissue or wound healing settings where spatial restriction modulates macrophage shape and signaling.
Overall, scaffold pore size is context-dependent, with the interplay between material properties (e.g., hydrogel vs. thermoplastic), macrophage source (e.g., BMDMs vs. tissue-resident macrophages), and biological environment (in vitro vs. in vivo) governing macrophage behavior. Future research should systematically compare these parameters across soft and hard tissue models to optimize scaffold design for targeted immune modulation.
Stiffness, ECM mimicry, and surface topography
Macrophage polarization can also be impacted by the scaffold cross-linking agent modulating stiffness. 1-ethyl-3-3-dimethyl aminopropyl carbodiimide (EDAC) cross-linked collagen networks have increased macrophages’ responsiveness to all inflammatory and anti-inflammatory cytokines. Hence, macrophages develop a profound anti-inflammatory effect in the presence of EDAC and anti-inflammatory cytokines[34].
Biomaterial scaffolds that mimic the native ECM are anti-inflammatory and lead to M2 macrophage polarization. Decellularized porcine brain matrixes are made from brain tissue with all cells removed, leaving only the ECM remaining. These matrices support cell adhesion, migration, and differentiation and promote tissue healing by preserving FGF-1 and FGF-2, enhancing macrophage polarization toward the M2 phenotype[35]. Another investigation showed that poly-L-lactide-co-polycaprolactone (PLA-PCL) electrospun matrices provide a high surface area and porosity, which alters macrophage shape and influences M2 polarization after the initial inflammatory phase. The combination of nano-topography that mimics the ECM and structural properties of the scaffold induces M2 macrophage polarization[36].
Luu et al. suggest that surface topology is predictive of macrophage polarization and tissue graft survival[37]. This group designed titanium surfaces with grooves ranging from 400-500 nm and conducted the in vitro study using mouse bone marrow cells. They found that micro- and nanopatterned grooves on the surface of biomaterials drove macrophages toward an anti-inflammatory M2 phenotype; however, they found no difference in immune activation. They also discovered that macrophages on intermediate groove widths had significantly increased expression of IL-10, an anti-inflammatory cytokine associated with M2 macrophage polarization, compared to other surfaces[37]. Other studies have demonstrated similar findings that the surface topography of biomaterials regulates macrophage response, impacting tissue regeneration and inflammation[38,39].
Surface chemistry and charge
Implant surface chemical properties such as charge, hydrophilicity, and the presence of different functional groups can induce polarization of macrophages and impact inflammatory response to foreign bodies. For example, Buck et al. investigated polystyrene surfaces containing various functional groups to determine which can induce M2 polarization [Table 1]. They found that carboxylic acid functional groups were the most effective in stimulating anti-inflammatory and M2 macrophage polarization and increasing factors promoting biomaterial integration, such as angiogenesis, integrin signaling, and cytokine signaling[40]. The presence of COOH groups enhanced the adsorption of integrin-binding proteins, likely promoting integrin-mediated signaling pathways. Additionally, the COOH functionalized surfaces were observed to increase the secretion of proteins associated with integrin and cytokine signaling and angiogenesis, fostering a microenvironment conducive to tissue repair and biomaterial integration. Bygd et al. conducted a similar study using poly (N-isopropylacrylamide-co-acrylic acid) nanoparticles modified with different functional groups[41] that could influence corresponding anti-inflammatory M2 macrophage polarization. They also found that an IL-10 low, TNF high (M1) phenotype is associated with ether, phosphonic acid, sulfone, and sulfonic acid functional groups[41]. Increasing nanoparticle hydrophilicity was also shown to significantly reduce foreign body inflammatory response and induce M2 macrophage polarization[14].
Summary of biomaterials and their key properties affecting macrophage polarization
Biomaterial | Properties | Effects |
Hydrogel-coated implants | ROS scavenging, promotes M2 polarization | Prevents biofilm formation, reduces inflammation, supports osseointegration |
Carbohydrate-functionalized nanoparticles | Targets macrophages, directs M2 polarization | Enhances tissue repair, reduces infections |
Oxidized glucomannan glycopeptide hydrogel | Mimics ECM, induces M2 polarization | Promotes BMSC differentiation, osteointegration |
Lanthanum-doped hydroxyapatite/chitosan scaffold | Upregulates SMAD pathway, anti-inflammatory | Enhances osteoblast differentiation, bone regeneration |
Bioactive glass-ceramic scaffold with molybdenum | Enhances mitochondrial function, induces M2 polarization | Improves periodontal wound healing, regenerates tissues |
Decellularized ECM hydrogel | Mimics native ECM, induces M2 polarization | Enhances fibroblast migration, accelerates wound healing |
Electrospun poly(l-lactide) nanofibers | Suppresses the JAK-STAT pathway, promotes M2 shift | Supports epidermal regeneration, neovascularization |
Thermosensitive hydrogel (Pluronic F-127 with Phillyrin) | Maintains hydration, promotes M2 polarization | Accelerates wound closure, promotes angiogenesis |
Magnesium-incorporated scaffolds | Enhances hydrophilicity, supports M2 polarization | Reduces inflammation, promotes vascularization |
Borosilicate glass scaffolds | pH-responsive, releases anti-inflammatory agents | Promotes M1 to M2 transition, supports tissue regeneration |
Implants can also be coated with bioactive materials to influence their interaction with the host tissue. Incorporation of magnesium in in vitro human macrophage culture and in vivo mice trials, for instance, have increased M2 macrophage polarization, as judged by an increased expression of CD163, a marker of M2 phenotype. The Mg-incorporated scaffolds displayed varying porosity, with lower doses producing higher porosity, which is correlated with M2 macrophage polarization. Another hypothesis the researchers used to explain this macrophage polarization difference is the charge interaction between the positively charged Mg and negatively charged fibrinogen scaffold, producing disordered structures associated with M2 polarization[42]. Non-reduced graphene oxide (GO) increases CD80, a surface marker for pro-inflammatory M1 macrophages. Thermally reduced graphene oxide (rGO) materials were not shown to cause the same increase in CD80, but they showed decreases in oxidative stress and pro-inflammatory cytokine secretion, promoting tissue regeneration. The mechanism of this material’s effect on the inflammatory response is poorly understood[43]. Synthetic fibrous glycopeptide hydrogel can mimic the natural ECM when attached to PCL/nano-hydroxyapatite (nHA) scaffolds. This structure induces proliferation and osteogenic differentiation of bone mesenchymal stem cells (BMSCs), promoting M2 macrophage polarization and inhibiting the inflammatory response to the bioengineered tissue.
The macrophage polarization was driven by mannose repeating units of the glycopeptide hydrogel binding to the mannose receptor and activating ERK/STAT6 signaling[38]. Polyethylene terephthalate (PET) and PET+ collagen biomaterials induce a pro-inflammatory response indicated by high inflammatory markers (TNF-α, IL-6, IL-1β, MCP-3, MIP-1α) and high M1/M2 indices. Polypropylene increases the expression of CCL18 and MDC, which are markers of fibrosis and anti-inflammatory M2 phenotype[44]. Palmer et al. explored the effects of scaffold materials on macrophage polarization using an in vivo rat model and found that unmodified PCL scaffolds demonstrated a more robust foreign body response and lack of macrophage adherence compared to poly(lactic-co-glycolic acid) (PLGA) and polyurethane (PUR) scaffolds[45]. PLGA scaffolds showed a more M1-dominant response, while PUR scaffolds showed a mixed M1 and M2 response[45]. Bacteroid cerium oxide particles on the surface of biomaterials effectively regulate NO and ROS, key signaling molecules in macrophage polarization. These particles facilitated a rapid shift from M1 to M2 macrophages, promoting early vascularization and bone regeneration around implants[46].
Surface coat materials can impact the immune response through different mechanisms and interactions with the host tissue. Molybdenum coating of scaffolds induces M2 macrophage polarization by enhancing mitochondrial function and causing a shift from glycolysis to OXPHOS[47]. Hyaluronic acid coating of collagen nanofibers increases the mechanical softness and shifts macrophages to an M2 phenotype by causing elongation. The M2 polarized macrophages then induced an anti-inflammatory response by recruiting endogenous progenitor cells to the wound site to promote tissue regeneration[48]. Ziegler et al. conducted a study using a combined allograft adipose matrix (AAM) and fascia matrix scaffold for adipose tissue engineering[49]. They found that this scaffold type induces the prodegenerative M2 macrophage phenotype and promotes angiogenesis, leading to tissue repair. Compared to the AAM scaffold, the combined 50/50 AAM/fascia scaffold with hyaluronic acid had a significantly lower M1/M2 ratio and promoted more adipogenesis. The fascia component of the scaffold aided in the neovascularization of the tissue, supporting graft survival[49]. Hydrogels with integrin binding sites such as α2β1 promote the expression of CD206 and IL-10, which correlate with M2 macrophage polarization[49]. Platelet lysate-rich plasma macroporous hydrogel (PLPMH) scaffold degrades gradually and releases sphingosine-1-phosphate (S1P) and growth factors (TBF-β, VEGF, PDGF-BB), which mediate macrophage recruitment and M2 polarization[50].
Drug loading
In addition to surface coat materials, tissue grafts can be loaded with drugs or cytokines to modulate the inflammatory response and increase tissue regeneration. Ursolic acid is a natural anti-inflammatory compound that induces M2 macrophage polarization and improves bone regeneration when loaded into a mesoporous hydroxyapatite/chitosan scaffold[51]. Implants coated with IL-4, an anti-inflammatory cytokine, show M2 polarization and modulation of the innate immune reaction, promoting biomaterial integration[52]. Borosilicate glass scaffolds have been designed to respond to pH and temperature, which can control the release of anti-inflammatory agents. Decreased pH due to inflammation triggers the scaffold to release more anti-inflammatory agents, promoting the transition from M1 to M2[53]. IFN-gamma/SrBG composite scaffolds have a time-dependent release of IFN-gamma in the early stages to encourage angiogenesis and M1 polarization, followed by Sr2+ from SrBG to induce a shift to M2 polarization for osteogenesis[51]. Molecular modifications can deliver cytokines to mediate macrophage polarization, release agents such as dexamethasone to suppress inflammation, and incorporate specific physicochemical properties to influence macrophage behavior[30,41]. Additionally, scaffolds engineered for sequential cytokine release, such as those delivering IL-4, reinforced M2 polarization and promoted BMSC differentiation via the TGF-β1/Smad pathway, demonstrating significant improvements in bone repair[2]. Depending on the application, these strategies improve biomaterial integration and promote outcomes such as enhanced bone repair[32].
Biological microenvironment and cellular crosstalk
Interactions with cells, biomaterials, and the ECM shape macrophage polarization. Fibroblast-macrophage communication influences macrophage behavior through monocyte chemoattractant protein-1 (MCP-1), a chemokine with potent inflammatory signaling. It is intimately involved in the in vivo cellular response to foreign material, the inhibition of macrophage cytokine release, and the activation of fibroblast cytokine release. Co-cultures, an in vitro substitute to model in vivo cell-cell interactions of macrophages and fibroblasts, show dramatically increased production of MCP-1. Co-culturing macrophages with fibroblasts increased MCP-1 secretion 2.5-fold, enhancing macrophage infiltration and fibroblast proliferation[54]. Co-culturing macrophages with fibroblasts increased MCP-1 secretion 2.5-fold, enhancing macrophage infiltration and fibroblast proliferation[54]. This interaction also led to the in vitro formation of multinucleated cells resembling foreign-body giant cells. The implications of this cell-cell interaction are still unknown but illustrate that macrophage behavior and polarization state are intrinsically tied to cytokine signals and other cells nearby[52,55]. Jeong et al. looked at cellular density in vitro wound environments and demonstrated that it modulates macrophage polarization; higher cell densities reduced inflammatory cytokine production. Jeong et al. looked at cellular density in in vitro wound environments and showed that it modulates macrophage polarization; higher cell densities reduced inflammatory cytokine production, favoring M2 polarization[55]. Hachim et al. assert that the interaction of MCP-1 warrants further examination of IL-4 coating upon other cells participating in the remodeling process, favoring M2 polarization[52].
APPLICATIONS OF MACROPHAGE POLARIZATION IN HEAD AND NECK RECONSTRUCTION
Bone regeneration and vascularization
Engineered scaffolds geared toward bone regeneration and osteointegration focus on inducing an M2, anti-inflammatory microenvironment. M2 macrophages release anti-inflammatory cytokines such as IL-10 and TGF-Beta to promote chondrogenesis, osteoblast proliferation, and tissue repair while inhibiting matrix degradation[18,33,50,53]. Most studies agree that M1 inflammatory macrophages play an early role critical to bone regeneration by inducing angiogenesis[33,46,56,57]. Thus, many scaffold designs are engineered to promote a quick M1 to M2 transition instead of completely inhibiting M1 macrophages. Zhu et al. used a Bacteroid Cerium Oxide scaffold in mouse in vitro and rabbit in vivo studies, which induced M1 innate immune response to release NO, promoting angiogenesis but limiting the release of ROS. The scaffold transitioned M1 macrophages into the M2 phenotype, inducing mesenchymal stem cell differentiation and bone regeneration[46]. Similarly, Luo et al. used an IFN-γ/SrBG composite scaffold that first releases IFN-γ to promote M1 differentiation and then a day later releases Sr2+ from SrBG to induce an M1 to M2 transition. In turn, many of these scaffolds have shown overall increased osteointegration[57]. Hu et al. used a 3D porous biodegradable scaffold that can improve luteolin’s bioactivity and release period, inhibiting PI3K-AKT-mTOR and AMPK-alpha-1 M1 polarization and activating the IL-10 M2 polarization[58]. The results increased luteolin bioavailability and stimulated new bone formation in critically sized bone defects[58]. Tian et al. designed a Dual stimulus-responsive borosilicate glass scaffold that inhibited mitochondrial fission, decreasing the release of ROS. The results also showed a significant increase in the M2 macrophage ratio after 4 weeks, stimulating the restoration of diabetic alveolar bone defects[53]. Chen et al. used a titanium implant using nanotubes with a double Chitosan hydrogel layer that first releases an IL-4 pro-inflammatory cytokine followed by IFN-γ release. The results showed an increased M2 anti-inflammatory microenvironment that improved chondroblast proliferation and osteogenesis in bone[18].
Soft tissue repair and infection control
Bioengineered tissue regenerating scaffolds increase soft tissue wound healing through a mechanism similar to osteointegration. Implants mimic the natural ECM to induce an M2 macrophage phenotype promoting anti-inflammatory mechanisms, which generates new soft tissue. He et al. emphasized using decellularized ECM and nanofibrous as the leading scaffold types in bioactive wound dressing for skin trauma[47]. Specifically, an ECM hydrogel derived from pericardium promoted an M2 phenotype that effectively induces fibroblast migration and angiogenesis. They also discussed how using an Ursolic acid-encapsulated nanofibrous wound dressing effectively stimulated M2 polarization, improving wound closure, re-epithelialization, revascularization, and hair follicle regeneration[51]. Xie et al. used electrospun poly(l-lactide) nanofibers that downregulate the JAK-STAT and NF-KB pathways, thus suppressing the activation of M1 macrophages and driving a shift toward M2 macrophages[59]. This also induces a morphological change that favors M2 polarization. These nanofiber models showed a decrease in M1 macrophage markers, TNF-alpha, and IL-6 and an increase in M2 macrophage markers, which promoted epidermal regeneration, collagen fiber deposition, and neovascularization. This was demonstrated in both in vitro and in vivo models[59]. Huang et al. designed a thermosensitive hydrogel soft tissue wound dressing from Pluronic F-127 and Phillyrin, which effectively promotes the transition from M1 to M2 macrophages, inducing keratinocyte migration, accelerated wound closure, and enhanced angiogenesis. The polymer hydrogels have a soft texture and good hydrophilicity but remain insoluble in water. These attributes, along with the porous structure of Pluronic F-127, resist infection, create a hydrated environment, and remove necrotic tissue[60].
Immunomodulation for long-term implant stability
Head and neck reconstructive surgeries frequently rely on materials such as titanium plates, silicone implants, hydroxyapatite, and polymer-based scaffolds to restore function and structure following trauma, cancer resection, or congenital disabilities. Reports have highlighted several complications associated with these materials, including postoperative infections, hardware extrusion, bone resorption, and malunion. Postoperative infections are particularly problematic in the context of intraoral and extraoral approaches, with rates reported at 5.9% and 10%, respectively[61]. These infections can destabilize implants through “bone-softening,” which compromises osseointegration[62]. Malunion, another reported complication, often arises from inadequate osseous reduction or improper stabilization. Fibrous malunion occurs when fractures fail to achieve sufficient osseous healing, leading to structural instability[62]. In a large study of mandibular reconstruction cases, malunion was observed in 1.3% of approximately 19,000 patients[63]. Additional challenges include hardware extrusion, bone resorption, and dystrophic calcifications, all interfering with implant longevity and performance[62,64]. In cancer-related facial skin reconstruction, necrosis of full-thickness grafts is a notable complication, with rates reported at 13.6%[65]. These findings underscore the need for biomaterials that support improved healing and long-term integration in complex reconstructive scenarios.
Modulating macrophage polarization has emerged as a clinically relevant strategy to mitigate complications such as infection, fibrosis, and poor osseointegration in head and neck reconstruction. By promoting a shift from the pro-inflammatory M1 phenotype to the tissue-regenerative M2 phenotype, bioengineered materials can enhance tissue integration, minimize inflammatory responses, and improve implant stability. This approach has been investigated in preclinical models and early-stage clinical research, particularly for applications such as mandibular reconstruction, dental implants, and skin grafting. Recent studies demonstrate that biomaterials with functionalized coatings, such as hydrogel-modified implants, can modulate macrophage behavior by scavenging ROS and driving M2 polarization. This prevents biofilm formation, reduces chronic inflammation, and supports osseointegration. For example, a hydrogel coating loaded with tannic acid-D-tyrosine nanoparticles has been shown to avoid biofilm formation and alleviate oxidative stress while promoting M2 formation[66]. In a rat femur infection model, this coating significantly reduced bacterial burden and improved implant integration by promoting M2 macrophage activity[66]. Similarly, carbohydrate-functionalized polymeric nanoparticles targeting macrophages have shown potential in redirecting macrophage polarization to M2, enhancing tissue repair, and reducing implant-associated infections[67].
Recent studies highlight additional strategies for clinically relevant immunomodulation. Dopamine-modified hyaluronan-dopamine hydrogels have been shown to regulate macrophage polarization via the NF-κB pathway, where lower dopamine concentrations promote M2 polarization and higher concentrations favor M1 macrophages, offering tunable control over immune responses[68]. Similarly, bone-mimicking PLA membranes containing hydroxyapatite and collagen sequentially induced M1 and M2 macrophage phenotypes in vitro, supporting immunomodulation in bone healing[69]. Hydrogels composed of polysaccharides or proteins have also demonstrated the ability to promote M2 polarization and enhance wound healing in in vitro models[70]. Moreover, scaffold geometry influences macrophage behavior, as honeycomb tricalcium phosphate scaffolds with larger pores promoted a mixed M1/M2 response, enhancing bone tissue formation[71].
Additional preclinical studies further confirm macrophage polarization’s role in improving biomaterials’ clinical performance. For instance, an oxidized glucomannan glycopeptide hydrogel (GRgel) coated on a 3D-printed PCL/nHA scaffold has been shown to promote M2 polarization in vivo[72]. This composite scaffold mimics the ECM and induces M2 macrophage polarization, creating an anti-inflammatory microenvironment conducive to BMSC differentiation and osteointegration. In a rat cranial defect model, the glycopeptide-coated scaffold achieved a bone healing efficiency of 83% ± 12% at 12 weeks post-operation, significantly outperforming the non-coated scaffold[72]. These findings highlight the translational potential of glycopeptide-based scaffolds in advancing head and neck reconstruction.
A related study demonstrated the efficacy of a magnetic lanthanum-doped hydroxyapatite/chitosan scaffold, which promoted an anti-inflammatory microenvironment by upregulating the SMAD 1/5/9 signaling pathway[73]. This scaffold enhanced osteoblast differentiation and bone regeneration in rat calvarial defects[73]. Additionally, a 3D-printed bioactive glass-ceramic scaffold incorporating molybdenum enhanced M2 polarization and accelerated periodontal wound healing in a periodontitis model[47]. This scaffold also stimulated the regeneration of bone, periodontal ligaments, and cementum tissue[47].
Despite promising preclinical data, there remains a lack of published clinical trials directly evaluating the effects of biomaterial-mediated macrophage polarization in head and neck reconstruction. Most current studies focus on animal models or in vitro systems, underscoring the need for translational research to assess the clinical relevance of these immunomodulatory strategies. Future clinical investigations will be essential to validate these findings and optimize biomaterial design for patient-specific applications.
Collectively, these biomaterials demonstrate the possibility of reducing postoperative complications, improving tissue integration, and improving overall outcomes in head and neck reconstructive procedures by exploiting their ability to modulate the inflammatory microenvironment, especially concerning macrophage polarization.
CONCLUSION
Macrophages play an integral role in wound healing, and recent advances in regenerative medicine have increasingly focused on modulating this important cell line through biomaterial engineering. Tailored biomaterials that influence macrophage polarization have shown promise in improving tissue regeneration and reducing implant-associated complications[74]. However, gaps remain in understanding macrophage interactions in complex tissue environments, particularly in the context of patient-specific immune variability. Continued progress in identifying and incorporating factors that influence macrophage behavior into novel biomaterials will enhance patients’ lives. Biomaterials are used in many head and neck reconstructive procedures; these patients, therefore, stand to benefit significantly from continued advancement in bioengineering.
Personalized biomaterial design is an emerging strategy to address these challenges. Future efforts should incorporate patient-specific immune profiling, including factors such as comorbidities and genetic predispositions, to develop scaffolds optimized for individual immune responses. For instance, 3D bioprinting and machine learning algorithms can be leveraged to generate more effective patient-specific implant architectures that modulate macrophage polarization. Incorporating immune-modulating coatings such as autologous proteins or cytokine delivery systems tailored to patient-specific inflammatory profiles could improve biomaterial integration.
In particular, the transition between M1 and M2 macrophage phenotypes remains a key area for future research. While some signaling pathways have been identified, the interactions between macrophages and other immune or stromal cells during wound healing remain poorly understood. Moreover, experimental approaches such as ex vivo models using patient-derived macrophages and single-cell RNA sequencing will be critical for understanding immune responses at a granular level. These models can provide data to fuel AI-based scaffold optimization, ensuring biomaterials are structurally and mechanically suited for head and neck reconstruction and immunologically compatible. For example, the mechanisms through which macrophages orchestrate vascularization, including initiating angiogenesis and stabilizing vascular networks, require further exploration. These processes are crucial for effective tissue regeneration and biomaterial integration[75].
Furthermore, biomaterials capable of delivering immunomodulatory signals in a temporally and spatially controlled manner represent a promising but underdeveloped area of research. Hydrogels, for instance, have shown potential as immunomodulatory scaffolds to guide macrophage behavior. However, their long-term effects on sustained M2 polarization need further validation in clinical applications[74]. Another challenge lies in developing biomaterials tailored to individual patient profiles, given the variability in immune responses associated with comorbidities such as diabetes or autoimmune disorders. Personalized strategies to design biomaterials that address this variability are essential for improving clinical outcomes.
Future research should prioritize these gaps by focusing on advanced biomaterials that regulate macrophage polarization, promote vascularization, and adapt to patient-specific immune responses. Additionally, integrating cutting-edge technologies, such as artificial intelligence, to optimize biomaterial design offers a pathway to accelerate innovations in regenerative medicine. By addressing these challenges, biomaterials can move closer to achieving their full potential in restoring tissue function and improving patient outcomes[5,74,75].
DECLARATIONS
Authors’ contributions
Conceptualization: Curry J, Palmer W, Bridgham K, Short S
Methodology and data curation: Samarah H. Jackson J
Formal analysis and writing - original draft: Samarah H, Jackson J, Kaplan Z, Nicodem M, Kumar P
Investigation: Samarah H, Jackson J
Visualization: Samarah H
Supervision: Curry J, Palmer W, Bridgham K, Short S, Harshyne L
Writing - review and editing: Samarah H, Jackson J, Curry J, Palmer W, Bridgham K, Short S., Harshyne L
Availability of data and materials
The data supporting this study’s findings are available from the corresponding author upon reasonable request. No publicly available datasets were used or generated during the current study.
Financial support and sponsorship
None.
Conflicts of interest
All authors declared that there are no conflicts of interest.
Ethical approval and consent to participate
Not applicable.
Consent for publication
Not applicable.
Copyright
© The Author(s) 2025.
REFERENCES
1. Tsouknidas A. Advancements in biomaterials for bioengineering and biotechnology. Int J Mol Sci. 2024;25:7840.
2. Smith TD, Nagalla RR, Chen EY, Liu WF. Harnessing macrophage plasticity for tissue regeneration. Adv Drug Deliv Rev. 2017;114:193-205.
3. Söhling N, Ondreka M, Kontradowitz K, Reichel T, Marzi I, Henrich D. Early immune response in foreign body reaction is implant/material specific. Materials. 2022;15:2195.
4. Castañón-Cortés LG, Bravo-Vázquez LA, Santoyo-Valencia G, et al. Current advances in the development of microRNA-integrated tissue engineering strategies: a cornerstone of regenerative medicine. Front Bioeng Biotechnol. 2024;12:1484151.
5. Gharibshahian M, Torkashvand M, Bavisi M, Aldaghi N, Alizadeh A. Recent advances in artificial intelligent strategies for tissue engineering and regenerative medicine. Skin Res Technol. 2024;30:e70016.
6. Jorgensen AM, Mahajan N, Atala A, Murphy SV. Advances in skin tissue engineering and regenerative medicine. J Burn Care Res. 2023;44:S33-41.
7. Blair NF, Frith TJR, Barbaric I. Regenerative medicine: advances from developmental to degenerative diseases. In: El-khamisy S, Editor. Personalised medicine. Cham: Springer International Publishing; 2017. pp. 225-39.
8. Yao Y, Xu XH, Jin L. Macrophage polarization in physiological and pathological pregnancy. Front Immunol. 2019;10:792.
9. BioRender: science illustration and figure creation tool. Available from: https://www.biorender.com/. [Last accessed on 16 Apr 2025].
10. Pang J, Maienschein-Cline M, Koh TJ. Monocyte/macrophage heterogeneity during skin wound healing in mice. J Immunol. 2022;209:1999-2011.
11. Jung SH, Hwang BH, Shin S, et al. Spatiotemporal dynamics of macrophage heterogeneity and a potential function of Trem2hi macrophages in infarcted hearts. Nat Commun. 2022;13:4580.
12. Lantz C, Radmanesh B, Liu E, Thorp EB, Lin J. Single-cell RNA sequencing uncovers heterogenous transcriptional signatures in macrophages during efferocytosis. Sci Rep. 2020;10:14333.
13. Mosquera JV, Auguste G, Wong D, et al. Integrative single-cell meta-analysis reveals disease-relevant vascular cell states and markers in human atherosclerosis. Cell Rep. 2023;42:113380.
14. Li W, Xu F, Dai F, et al. Hydrophilic surface-modified 3D printed flexible scaffolds with high ceramic particle concentrations for immunopolarization-regulation and bone regeneration. Biomater Sci. 2023;11:3976-97.
15. Krzyszczyk P, Schloss R, Palmer A, Berthiaume F. The role of macrophages in acute and chronic wound healing and interventions to promote Pro-wound healing phenotypes. Front Physiol. 2018;9:419.
16. Zhu Z, Ding J, Ma Z, Iwashina T, Tredget EE. Systemic depletion of macrophages in the subacute phase of wound healing reduces hypertrophic scar formation. Wound Repair Regen. 2016;24:644-56.
17. Kwak G, Cheng J, Kim H, et al. Sustained exosome-guided macrophage polarization using hydrolytically degradable PEG hydrogels for cutaneous wound healing: identification of key proteins and MiRNAs, and sustained release formulation. Small. 2022;18:e2200060.
18. Chen J, Li M, Yang C, et al. Macrophage phenotype switch by sequential action of immunomodulatory cytokines from hydrogel layers on titania nanotubes. Colloids Surf B Biointerfaces. 2018;163:336-45.
19. Carnicer-Lombarte A, Chen ST, Malliaras GG, Barone DG. Foreign body reaction to implanted biomaterials and its impact in nerve neuroprosthetics. Front Bioeng Biotechnol. 2021;9:622524.
20. Cha BH, Shin SR, Leijten J, et al. Integrin-mediated interactions control macrophage polarization in 3D hydrogels. Adv Healthc Mater. 2017;6:1700289.
21. Pang QM, Yang R, Zhang M, et al. Peripheral blood-derived mesenchymal stem cells modulate macrophage plasticity through the IL-10/STAT3 pathway. Stem Cells Int. 2022;2022:5181241.
22. Nie Z, Fan Q, Jiang W, et al. Placental mesenchymal stem cells suppress inflammation and promote M2-like macrophage polarization through the IL-10/STAT3/NLRP3 axis in acute lung injury. Front Immunol. 2024;15:1422355.
23. Waqas SFH, Ampem G, Röszer T. Analysis of IL-4/STAT6 signaling in macrophages. In: Badr MZ, Editor. Nuclear receptors. New York: Springer; 2019. pp. 211-24.
24. D’Alessio FR, Craig JM, Singer BD, et al. Enhanced resolution of experimental ARDS through IL-4-mediated lung macrophage reprogramming. Am J Physiol Lung Cell Mol Physiol. 2016;310:L733-46.
25. Daniel B, Nagy G, Horvath A, et al. The IL-4/STAT6/PPARγ signaling axis is driving the expansion of the RXR heterodimer cistrome, providing complex ligand responsiveness in macrophages. Nucleic Acids Res. 2018;46:4425-39.
26. Huang SC, Smith AM, Everts B, et al. Metabolic reprogramming mediated by the mTORC2-IRF4 signaling axis is essential for macrophage alternative activation. Immunity. 2016;45:817-30.
27. Spiller KL, Nassiri S, Witherel CE, et al. Sequential delivery of immunomodulatory cytokines to facilitate the M1-to-M2 transition of macrophages and enhance vascularization of bone scaffolds. Biomaterials. 2015;37:194-207.
28. Wu J, Jiang L, Wang S, Peng L, Zhang R, Liu Z. TGF β1 promotes the polarization of M2-type macrophages and activates PI3K/mTOR signaling pathway by inhibiting ISG20 to sensitize ovarian cancer to cisplatin. Int Immunopharmacol. 2024;134:112235.
29. Gaharwar AK, Singh I, Khademhosseini A. Engineered biomaterials for in situ tissue regeneration. Nat Rev Mater. 2020;5:686-705.
30. Liu Y, Suarez-Arnedo A, Riley L, Miley T, Xia J, Segura T. Spatial confinement modulates macrophage response in microporous annealed particle (MAP) scaffolds. Adv Healthc Mater. 2023;12:e2300823.
31. Wang X, Fu X, Luo D, et al. 3D printed high-precision porous scaffolds prepared by fused deposition modeling induce macrophage polarization to promote bone regeneration. Biomed Mater. 2024;19:035006.
32. Li W, Dai F, Zhang S, et al. Pore size of 3D-printed polycaprolactone/polyethylene glycol/hydroxyapatite scaffolds affects bone regeneration by modulating macrophage polarization and the foreign body response. ACS Appl Mater Interfaces. 2022;14:20693-707.
33. Mahon OR, Browe DC, Gonzalez-Fernandez T, et al. Nano-particle mediated M2 macrophage polarization enhances bone formation and MSC osteogenesis in an IL-10 dependent manner. Biomaterials. 2020;239:119833.
34. Sridharan R, Ryan EJ, Kearney CJ, Kelly DJ, O’Brien FJ. Macrophage polarization in response to collagen scaffold stiffness is dependent on cross-linking agent used to modulate the stiffness. ACS Biomater Sci Eng. 2019;5:544-52.
35. Hong JY, Seo Y, Davaa G, Kim HW, Kim SH, Hyun JK. Decellularized brain matrix enhances macrophage polarization and functional improvements in rat spinal cord injury. Acta Biomater. 2020;101:357-71.
36. Pisani S, Dorati R, Genta I, Benazzo M, Conti B, Prina Mello A. A study focused on macrophages modulation induced by the polymeric electrospun matrices (EL-Ms) for application in tissue regeneration: in vitro proof of concept. Int J Pharm. 2021;603:120712.
37. Luu TU, Gott SC, Woo BW, Rao MP, Liu WF. Micro- and nanopatterned topographical cues for regulating macrophage cell shape and phenotype. ACS Appl Mater Interfaces. 2015;7:28665-72.
38. Wang M, Chen F, Tang Y, et al. Regulation of macrophage polarization and functional status by modulating hydroxyapatite ceramic micro/nano-topography. Mater Des. 2022;213:110302.
39. Zheng X, Chen L, Tan J, et al. Effect of micro/nano-sheet array structures on the osteo-immunomodulation of macrophages. Regen Biomater. 2022;9:rbac075.
40. Buck E, Lee S, Stone LS, Cerruti M. Protein adsorption on surfaces functionalized with COOH groups promotes anti-inflammatory macrophage responses. ACS Appl Mater Interfaces. 2021;13:7021-36.
41. Bygd HC, Forsmark KD, Bratlie KM. Altering in vivo macrophage responses with modified polymer properties. Biomaterials. 2015;56:187-97.
42. Bessa-Gonçalves M, Ribeiro-Machado C, Costa M, et al. Magnesium incorporation in fibrinogen scaffolds promotes macrophage polarization towards M2 phenotype. Acta Biomater. 2023;155:667-83.
43. Cicuéndez M, Casarrubios L, Barroca N, et al. Benefits in the macrophage response due to graphene oxide reduction by thermal treatment. Int J Mol Sci. 2021;22:6701.
44. Grotenhuis N, Vd Toom HF, Kops N, et al. In vitro model to study the biomaterial-dependent reaction of macrophages in an inflammatory environment. Br J Surg. 2014;101:983-92.
45. Palmer JA, Abberton KM, Mitchell GM, Morrison WA. Macrophage phenotype in response to implanted synthetic scaffolds: an immunohistochemical study in the rat. Cells Tissues Organs. 2014;199:169-83.
46. Zhu S, Chen Y, Lu Z, et al. Bacteroid cerium oxide particles promote macrophage polarization to achieve early vascularization and subsequent osseointegration around implants. Biochem Biophys Res Commun. 2024;703:149647.
47. He XT, Li X, Zhang M, et al. Role of molybdenum in material immunomodulation and periodontal wound healing: targeting immunometabolism and mitochondrial function for macrophage modulation. Biomaterials. 2022;283:121439.
48. Niu Y, Stadler FJ, Yang X, Deng F, Liu G, Xia H. HA-coated collagen nanofibers for urethral regeneration via in situ polarization of M2 macrophages. J Nanobiotechnology. 2021;19:283.
49. Ziegler ME, Khabaz K, Khoshab N, et al. Combining allograft adipose and fascia matrix as an off-the-shelf scaffold for adipose tissue engineering stimulates angiogenic responses and activates a proregenerative macrophage profile in a rodent model. Ann Plast Surg. 2023;91:294-300.
50. Pan X, Yuan S, Xun X, et al. Long-term recruitment of endogenous M2 macrophages by platelet lysate-rich plasma macroporous hydrogel scaffold for articular cartilage defect repair. Adv Healthc Mater. 2022;11:e2101661.
51. Yu X, Wang Y, Liu X, Ge Y, Zhang S. Ursolic acid loaded-mesoporous hydroxylapatite/chitosan therapeutic scaffolds regulate bone regeneration ability by promoting the M2-type polarization of macrophages. Int J Nanomedicine. 2021;16:5301-15.
52. Hachim D, LoPresti ST, Yates CC, Brown BN. Shifts in macrophage phenotype at the biomaterial interface via IL-4 eluting coatings are associated with improved implant integration. Biomaterials. 2017;112:95-107.
53. Tian P, Zhao L, Kim J, et al. Dual stimulus responsive borosilicate glass (BSG) scaffolds promote diabetic alveolar bone defectsrepair by modulating macrophage phenotype. Bioact Mater. 2023;26:231-48.
54. Holt DJ, Chamberlain LM, Grainger DW. Cell-cell signaling in co-cultures of macrophages and fibroblasts. Biomaterials. 2010;31:9382-94.
55. Jeong JH, Hur SS, Lobionda S, et al. Heparin-mimicking polymer-based hydrogel matrix regulates macrophage polarization by controlling cell adhesion. Biochem Biophys Res Commun. 2023;642:154-61.
56. Xool-Tamayo J, Arana-Argaez VE, Villa-de la Torre F, Chan-Zapata I, Vargas-Coronado RF, Cauich-Rodríguez JV. Macrophages morphology and cytokine reeducation by ex situ copper thiol complexes. Immunopharmacol Immunotoxicol. 2024;46:20-32.
57. Luo M, Zhao F, Liu L, et al. IFN-γ/SrBG composite scaffolds promote osteogenesis by sequential regulation of macrophages from M1 to M2. J Mater Chem B. 2021;9:1867-76.
58. Hu Y, Tang L, Wang Z, et al. Inducing in situ M2 macrophage polarization to promote the repair of bone defects via scaffold-mediated sustained delivery of luteolin. J Control Release. 2024;365:889-904.
59. Xie J, Wu X, Zheng S, Lin K, Su J. Aligned electrospun poly(L-lactide) nanofibers facilitate wound healing by inhibiting macrophage M1 polarization via the JAK-STAT and NF-κB pathways. J Nanobiotechnology. 2022;20:342.
60. Huang C, Teng J, Liu W, Wang J, Liu A. Modulation of macrophages by a phillyrin-loaded thermosensitive hydrogel promotes skin wound healing in mice. Cytokine. 2024;177:156556.
61. Shah A, Perez-Otero S, Tran D, Aponte HA, Oh C, Agrawal N. Infection rates of an intraoral versus extraoral approach to mandibular fracture repairs are equal: a systematic review and meta-analysis. J Oral Maxillofac Surg. 2024;82:449-60.
62. Perez D, Ellis E 3rd. Complications of mandibular fracture repair and secondary reconstruction. Semin Plast Surg. 2020;34:225-31.
63. Lander DP, Lee JJ, Kallogjeri D, et al. The impact of treatment delay on malunion and nonunion after open reduction of mandible fractures. Facial Plast Surg Aesthet Med. 2021;23:460-6.
64. Ginat D. Surgical implants in the head and neck: normal and abnormal imaging appearances. Semin Roentgenol. 2023;58:290-300.
65. Mamsen FPW, Kiilerich CH, Hesselfeldt-Nielsen J, et al. Risk stratification of local flaps and skin grafting in skin cancer-related facial reconstruction: a retrospective single-center study of 607 patients. J Pers Med. 2022;12:2067.
66. Ding Y, Liu G, Liu S, et al. A multifunction hydrogel-coating engineered implant for rescuing biofilm infection and boosting osseointegration by macrophage-related immunomodulation. Adv Healthc Mater. 2023;12:e2300722.
67. Andrade RGD, Reis B, Costas B, Lima SAC, Reis S. Modulation of macrophages M1/M2 polarization using carbohydrate-functionalized polymeric nanoparticles. Polymers. 2020;13:88.
68. Pei D, Zeng Z, Geng Z, et al. Modulation of macrophage polarization by secondary cross-linked hyaluronan-dopamine hydrogels. Int J Biol Macromol. 2024;270:132417.
69. Özcolak B, Erenay B, Odabaş S, Jandt KD, Garipcan B. Effects of bone surface topography and chemistry on macrophage polarization. Sci Rep. 2024;14:12721.
70. Giri PS, Rath SN. Macrophage polarization dynamics in biomaterials: implications for in vitro wound healing. ACS Appl Bio Mater. 2024;7:2413-22.
71. Takabatake K, Tsujigiwa H, Nakano K, et al. Effect of scaffold geometrical structure on macrophage polarization during bone regeneration using honeycomb tricalcium phosphate. Materials. 2024;17:4108.
72. Wang Y, Wang J, Gao R, et al. Biomimetic glycopeptide hydrogel coated PCL/nHA scaffold for enhanced cranial bone regeneration via macrophage M2 polarization-induced osteo-immunomodulation. Biomaterials. 2022;285:121538.
73. Wang Q, Tang Y, Ke Q, et al. Magnetic lanthanum-doped hydroxyapatite/chitosan scaffolds with endogenous stem cell-recruiting and immunomodulatory properties for bone regeneration. J Mater Chem B. 2020;8:5280-92.
74. Di Francesco D, Talmon M, Carton F, Fresu LG, Boccafoschi F. Macrophage polarization guided by immunomodulatory hydrogels. Hydrogels for tissue engineering and regenerative medicine. Elsevier; 2024. pp. 765-82.
Cite This Article

How to Cite
Download Citation
Export Citation File:
Type of Import
Tips on Downloading Citation
Citation Manager File Format
Type of Import
Direct Import: When the Direct Import option is selected (the default state), a dialogue box will give you the option to Save or Open the downloaded citation data. Choosing Open will either launch your citation manager or give you a choice of applications with which to use the metadata. The Save option saves the file locally for later use.
Indirect Import: When the Indirect Import option is selected, the metadata is displayed and may be copied and pasted as needed.
About This Article
Special Issue
Copyright
Data & Comments
Data
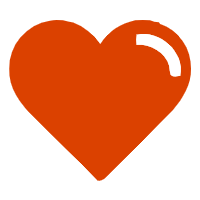
Comments
Comments must be written in English. Spam, offensive content, impersonation, and private information will not be permitted. If any comment is reported and identified as inappropriate content by OAE staff, the comment will be removed without notice. If you have any queries or need any help, please contact us at support@oaepublish.com.